INTRODUCTION
The current livestock system has concerns about environmental pollution, sustainability, and animal welfare as a whole [1]. The livestock industry must produce high-quality, low-cost meat in large quantities through environmentally sound, socially responsible and economically viable production systems [2]. The concept of cultured meat is proposed to produce meat without slaughtering livestocks by producing muscle tissues from muscle-derived stem cells in vitro. Cultured meat is the production of meat from animal cells based on the growth and recovery mechanism of animal muscle tissues [1]. After biopsy is taken from living animals, cultured meat can be achieved by separating stem cells from animal muscle biopsy samples and proliferating muscle cells in an environment that provides the energy and nutrients needed for cells to grow inside the animal [1]. Cultured meat has not yet become popular, but cultured meat has several distinct advantages compared to other types of alternative proteins. Cultured meat has the potential to solve these problems. Cultured meat production, once optimized, will produce more meat using fewer resources [3] and could provide cheap sources of rich minerals, vitamins, fats, and amino acids [4]. In Europe, cultured meat was found to have about 82%–96% lower water use, 99% lower land use, and 78%–96% lower greenhouse gas emissions compared to most traditional produced livestock systems [5]. Cultured meat has great potential to eliminate animal suffering and sacrifice during slaughter. In addition, since cells are supplied without slaughter, the pain of slaughter can be eliminated. Cultured meat can be an attractive option for vegetarians, vegans, and opponents who refuse to consume meat for ethical reasons [6]. The number of animals required for cell culture is smaller than that for general meat production [7]. In addition, cultured meat can achieve intensive agricultural reduction without requiring the transport of live animals to slaughter, thus preventing the exponential spread of animal disease [8]. Cultured meat has the potential to significantly reduce animal suffering while meeting all nutritional and hedonic requirements of eating meat [6].
For cultured meat to be used as a reliable alternative to livestock meat, laboratory or factory-grown meat must be produced efficiently. They also must mimic all physical senses such as visual appearance, smell, texture, and, of course, taste of traditional meat [9]. Imitation and efficiency are two key requirements for meat alternatives to be accepted and industrialized [1]. Various types of cells can be used for cultured meat. Pluripotent stem cells and multipotent progenitor cells can also differentiate into muscle cells or fat cells, but they do not differentiate as efficiently as muscle satellite cells and must undergo rigorous safety testing [10]. For this reason, in order to obtain muscle satellite cells repeatedly, muscle tissue must be collected from donor animals.
In the process of producing cultured meat, the choice of animals as stem cell donors has a great influence on efficiency [11]. Since the yield of isolated muscle stem cells depends on conditions of the donor animal, several factors should be considered for more efficient satellite cell separation before selecting the donor animal [1]. The selection of cell donors can have a dramatic impact on the efficiency of the entire process [12]. Thus, it is important to identify the defining characteristics of optimal cell donors. What should be optimized for donor identification includes the following: 1) intensity of proliferation and differentiation; and 2) the lifespan of stem cells. In other words, the ability to continue to multiply while maintaining the ability to differentiate to form mature tissues for meat is important. Donor characteristics for the efficiency of cultured meat should focus on the harvest, efficiency, and quality of muscle satellite cells [12]. Various characteristics of the donor can affect the yield and quality of satellite cells. Melzener et al. [13] compared bovine satellite cells isolated from five breeds (Simmental, Limousin, Galloway, Holstein Friesian, and Belgian Blue), and as a result, satellite cells from Limousin and Belgian Blue cattle showed longer retention of differentiation capacity over long term passaging. The proliferation capacity of satellite cells decreases with donor age and varies with donor breeds and disease status [14]. There are major genetic and phenotypic differences between breeds. Certain characteristics, such as maximum size and weight gain rates, are selectively bred to optimize meat and milk production [12]. The carcass properties of cattle are greatly influenced by breeds [15]. Numerous studies have suggested that many aspects of muscle mass and meat quality are closely related to muscle fiber characteristics [16], and there is evidence that differences in muscle fiber characteristics between cattle breeds and cattle are already evident at birth [17]. This can be said that animal breeds affect muscle fiber characteristics, and furthermore, muscle mass and meat quality. In this study, three breeds of cattle mainly used in the Korean livestock industry were compared. A study was conducted to evaluate the suitability of three different breeds of cattle as donor animals for the production of cultured beef based on the proliferation, differentiation, and taste of cell culture.
MATERIALS AND METHODS
Satellite cells from Jeju black cattle (Bos taurus coreanae) used in the experiment were obtained from semimembranosus muscles of a 41-month-old black cattle at Namwon-eup, Seogwipo-si, Jeju-do, Korea. Satellite cells from Hanwoo (breed of cattle native to Korea; Bos Taurus coreanae) used in the experiment were obtained from the semimembranosus muscle of a 34-month-old Hanwoo at Farmstory Hannaeng placed in Eumseong-gun, Chungcheongbuk-do, Korea. Satellite cells of Holstein (Bos taurus taurus) used in the experiment were obtained from the semimembranosus muscle of a 16-month-old Holstein at Chungbuk University located in Cheongju-si, Chungcheongbuk-do, Korea. The animal study protocol was approved by the Institutional Animal Care and Use Committees of Chungbuk National University (CBNUR-1442-20). Tissues were collected from all cattle according to the shipping date.
Cattle satellite cells were isolated from tissues of each cattle breeds. After washing muscles in alcohol and sterile water, debris attached to these muscles were removed. At 37°C, collagenase type II (600 units/mL) is used to digest cattle muscle fibers in DMEM (11995-065, Gibco, Waltham, MA, USA) supplement with 3% penicillin-streptomycin-amphotericin B solution antibiotics (PSA, Lonza, Basel, Switzerland). To extract cells, muscle satellite cells were separated from muscles and centrifuged. The cells were filtered using a 100 µm cell strainer (431752, Corning®, USA), then a 40 µm cell strainer (431750, Corning, New York, NY, USA). Until the experiment, harvested muscle satellite cells were kept in liquid nitrogen in cell culture freezing medium (Gibco).
Cells for FACS were grown in a humidified incubator (5% CO2, 37°C) for 5 days on bovine collagen type I (A1064401, Gibco) coated flasks. Cells were suspended in FACS buffer (1:100 bovine serum albumin [BSA] in phosphate buffered saline [PBS]) and stained with APC anti-human CD29 antibody (303008, BioLegend, San Diego, CA, USA), PE-CyTM7 anti-human CD56 (335826, BD, Franklin Lakes, NJ, USA), FITC anti-sheep CD31 (MCA1097GA, Bio-Rad, Hercules, CA, USA), and FITC anti-sheep CD45 (MCA2220GA, Bio-Rad). After antibody incubation, cells were washed multiple times with 1X PBS and repaced to Ham’s F-10 nutrient mix (11550043, Gibco) with 1% PSA antibiotics and 20% fetal bovine serum (FBS; 2335015CV, Corning). Satellite cells were sorted by filtering for the CD31/CD45-, and CD29+/CD56+ populations.
A flask coated with bovine collagen type I (A1064401, Gibco) for proliferation. Add the collagen coating solution to the flask, and placed in an incubator at 37°C for a minimum of 16 hours. It was then dried in preparation for use in the experiment. Muscle satellite cells sorted using FACS were grown on flask coated with collagen in Ham’s F-10 medium (11550043, Gibco) containing 20% FBS (16000-044, Gibco), 1% PSA (17-745E, Lonza) and 5 ng/mL basic fibroblast growth factor (bFGF, 13256029, Gibco) as growth medium. Satellite cells were sown at a density of 1,800 cells/cm2 and grown at 37°C with 5% CO2. Cells were passaged every 6 days.
For muscle satellite cell differentiation, flasks were coated with Matrigel (354234, Corning). Cold 1X PBS at a 1:200 ratio was used to make matrigel coating solution. Add the collagen matrigel solution to the flask, and placed in an incubator at 37°C for a minimum of 4 hours. Following removal of the Matrigel coating solution, washed once with 1X PBS. The medium for cell proliferation was the same as the medium for proliferation mentioned above. Satellite cells were sown at a density of 1,800 cells/cm2 and changed differentiation medium (DMEM supplemented with 2% FBS and 1% PSA) every 6 days. Cells were grown with 5% CO2 at 37°C for 5 days.
In a coated 96-well plate, the satellite cells were seeded at a density of 5,000 cells/cm2 and cultivated for 5 or 6 days at 37°C with 5% CO2. Proliferated or differentiated cells were then washed with 1X PBS after the culture medium was removed. Additionally, cells were exposed to 2% paraformaldehyde (PFA; in PBS) for 45 minutes at 37°C. After washing multiple times with 1X PBS, cells were permeabilized with 0.1% Triton X-100 (in PBS) for 20 minutes at room temperature. Cells were then blocked with 2% BSA for 30 minutes at room temperature, followed by two rounds of washing with 1X PBS. Cell were treated with primary antibody for overnight period at 4°C in 2% BSA (in 0.1% Triton X-100). The primary antibodies recognizing paired box 7 (PAX7; PA5-68506, Invitrogen, Waltham, MA, USA) and myogenic differentiation (MYOD; bs-2442R, Bioss, Woburn, MA, USA) and monoclonal anti-myosin (M4276, Sigma-Aldrich, St. Louis, MO, USA). Cells were treated with a secondary antibody at room temperature for 2 hours after being washed multiple times with 1X PBS. Goat anti-Rabbit IgG (H + L) Cross-Adsorbed Secondary Antibody, Alexa Fluor 488 (A21121, Invitrogen) and goat anti-mouse IgG1 cross-adsorbed secondary antibody, Alexa Fluor 488 (A11008, Invitrogen) were used as the secondary antibodies. Finally, Hoechst 33342 reagent (1:2000, H3570, Invitrogen) was added. EVOS-5000 optical microscope was used to examine muscle satellite cells that were Immunofluorescence stained. Using the ImageJ program, skilled experts counted the number of muscle cell nuclei on imaging data.
The satellite cells were sown at a density of 1,800 cells/cm2 in a coated flask and grown at 37°C with 5% CO2 for 5 or 6 days. After removing the cultured medium, proliferated or differentiated cells washed 1X PBS. Muscle satellite cell samples were harvest using cell scrapers. RNA was extracted using the TRIzol reagent. According to the instructions provided by manufacturer, cDNA was created using a Reverse Transcription Master Premix (ELPIS-BIOTECH, Daejeon, Korea) with a template RNA Primer Mixture and 1.0 µg of mRNA as a template, followed by incubation at 60°C for 60 minutes and 94°C for 5 minutes. Expression levels of associated genes were examined by quantitative real-time polymerase chain reaction (RT-qPCR). For the examination of expression level, the gene glyceraldehyde-3-phospate dehydrogenase (GAPDH) was employed as an internal control. 1 µl of cDNA, 10 µl EzAmp™ FAST qPCR 2X Master Mix (ELPIS-BIOTECH), and 1 µl of each primer made up the 20 µl RT-qPCR reaction. After Amplification at 95°C for 10 minutes, 40 cycles of 95°C for 10 seconds and 61°C for 20 seconds were perfomed. Table 1 shows the sequences of primers used in RT-qPCR assays.
All measurements were repeated at least three times. A statistical processing program SAS (9.4 for Windows, SAS Institute, Cary, NC, USA) was used to test the significance of results. To compare significant differences between measured values, Duncan multiple range test was performed at a significance level of p < 0.05.
RESULTS AND DISCUSSION
To examine the suitability of different cattle breeds as donors for the generation of cultured meat, only satellite cells were extracted. Semimembranosus muscle biopsy samples from each breed have been separated into satellite cells. Satellite cells were extracted using the FACS method. A distinct population of satellite cells (with a CD31-, CD45-, CD29+, and CD56+ immunophenotype) was seen in all three breeds (Fig. 1).
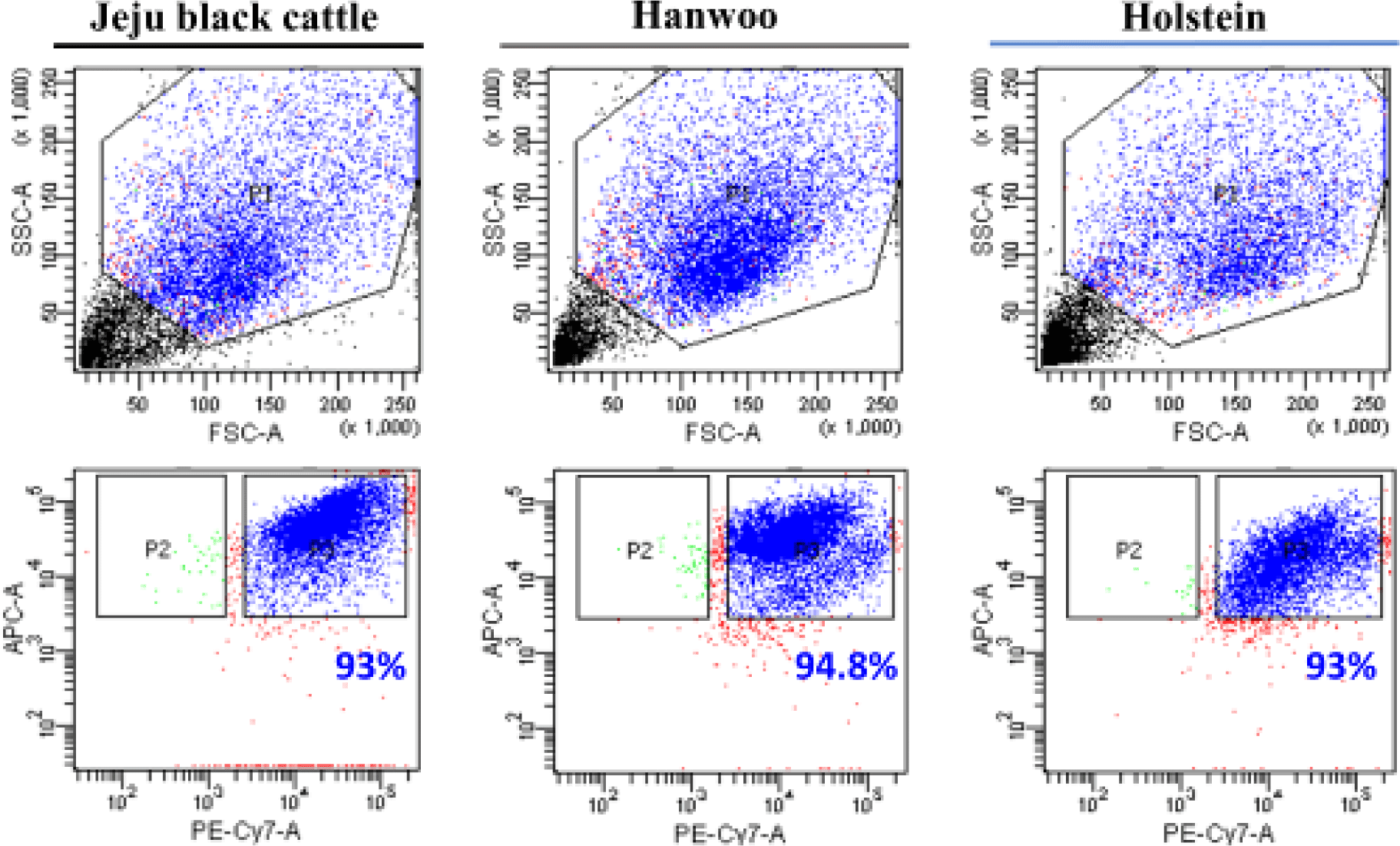
To determine the proliferation tendency of muscle satellite cells from three breeds of cattle, microscopic photographic analysis was performed (Fig. 2). After 1,800 cells per cm2 were seeded in a collagen-coated flask and cultured at 37°C for 6 days, satellite cells were subcultured from passage 4 to passage 12. The observed proliferation rate tended to decrease with long-term subculture.
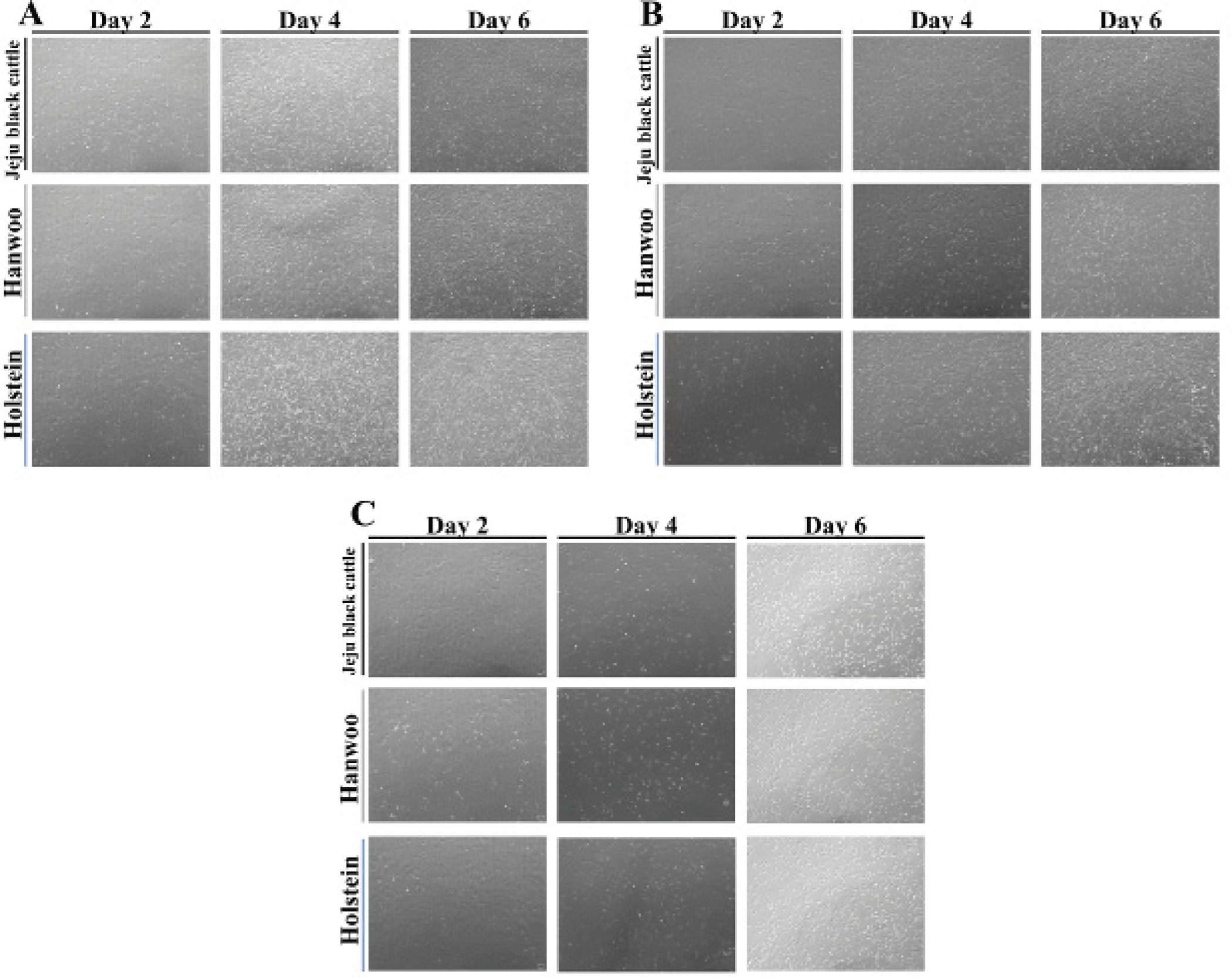
To determine the proliferation capacity of satellite cells from three breeds of cattle, the number of nuclei was measured on day 6 of passages 4, 8, and 12 by immunofluorescence staining with Hoechst 33342. In the case of passages 4 and 8, it was confirmed that cells were confluent on the flask on day 6 of proliferation (Fig. 3B). The same result was obtained when the nucleus was immunofluorescence stained (Fig. 3A). On the other hand, in the case of passage 12, cells on day 6 of proliferation tended not to be confluent on the flask. These results showed the same tendency as the number of nuclei in a graph (Fig. 3C). Overall, as the subculture progressed, the number of nuclei decreased, with passage 12 showing a rapid decrease in the number of nuclei.
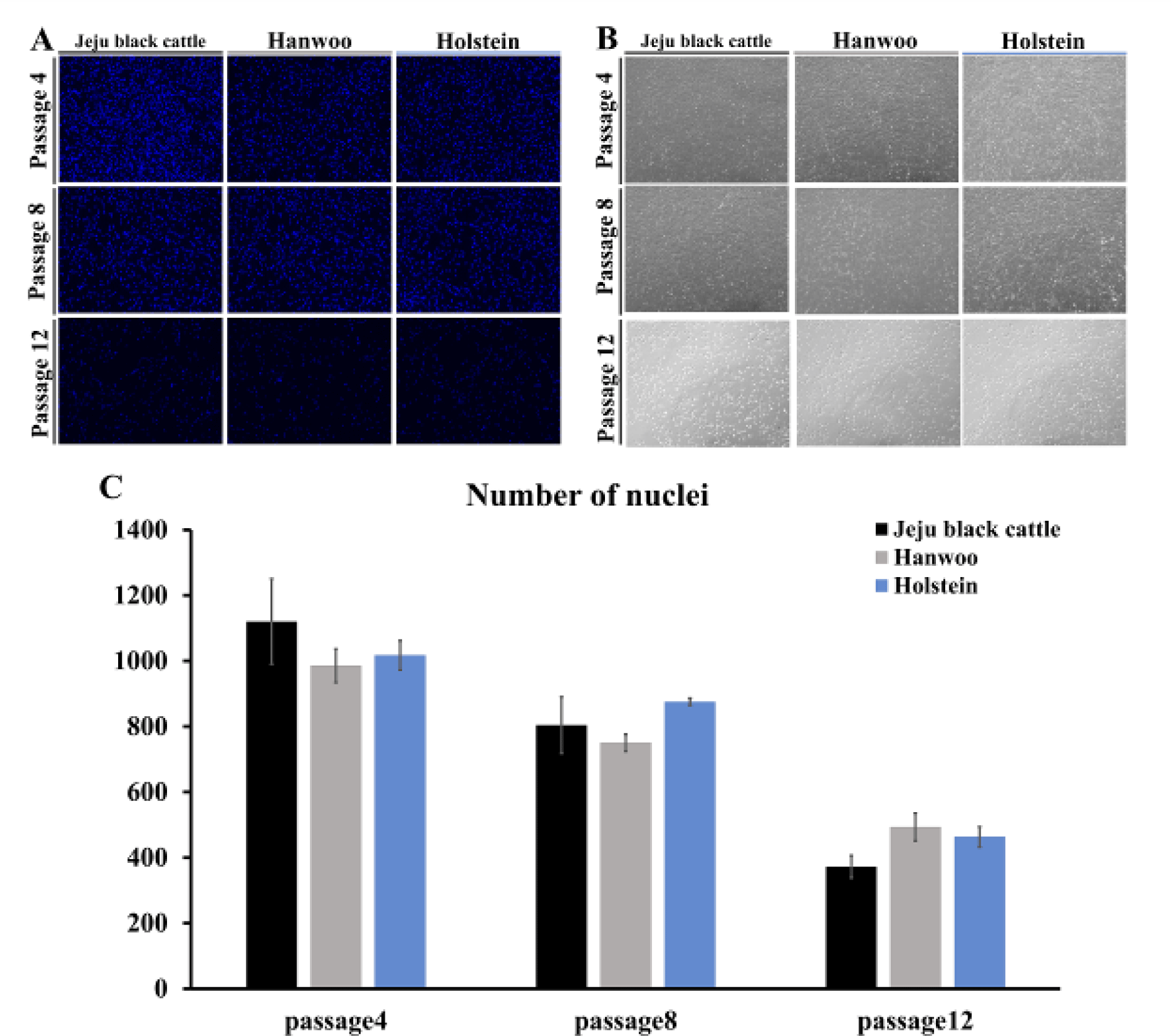
PAX7 is expressed in a stationary satellite cell state. Activated satellite cells also maintain PAX7 expression. After the cell cycle is stopped, activated muscle satellite cells undergo differentiation or self-regeneration. PAX7 upregulated muscle satellite cells can reacquire a stationary undifferentiated state [18], which means that muscle satellite cells can continue to proliferate through self-proliferation [19]. Immunofluorescence staining and RT-qPCR of the PAX7 factor were performed to confirm the identification and ability of muscle satellite cells to proliferate. Muscle satellite cells from three breeds of cattle were cultured under the same culture conditions. Experiments were conducted for muscle satellite cells on day 6 of proliferation for passages 4, 8, and 12. It was confirmed that cells proliferated were muscle satellite cells based on PAX7 immunofluorescence staining (Fig 4A, 4B, and 4C). Overall, PAX7 expression in muscle satellite cells from three breeds of cattle tended to decrease during subculture (Fig. 4D). Thus, the ability of muscle satellite cells to proliferate in passage 12 was greatly reduced. It was found that as the subculture progressed, the cell proliferation power decreased, the expression of PAX7 decreased, and the ability of muscle satellite cells to proliferate was not activated. Comparing PAX7 expression in satellite cells between breeds, Holstein breed showed higher PAX7 expression in passage 4 and passage 8 (Fig. 4D). Hanwoo muscle satellite cells showed significantly lower expression of PAX7 in passage 4 (p < 0.05). However, unlike other breeds, it showed no decrease in expression of PAX7 when cultivated up to passage 8. Thus, its proliferation power was maintained. In passage 12, there was no discernible difference in the three types of muscle satellite cells’ capacity for proliferation (Fig. 4D). Overall, considering all passages, the PAX7 expression level of Holstein is higher than that of the other two breeds, so it is estimated that the proliferation potential is also high.
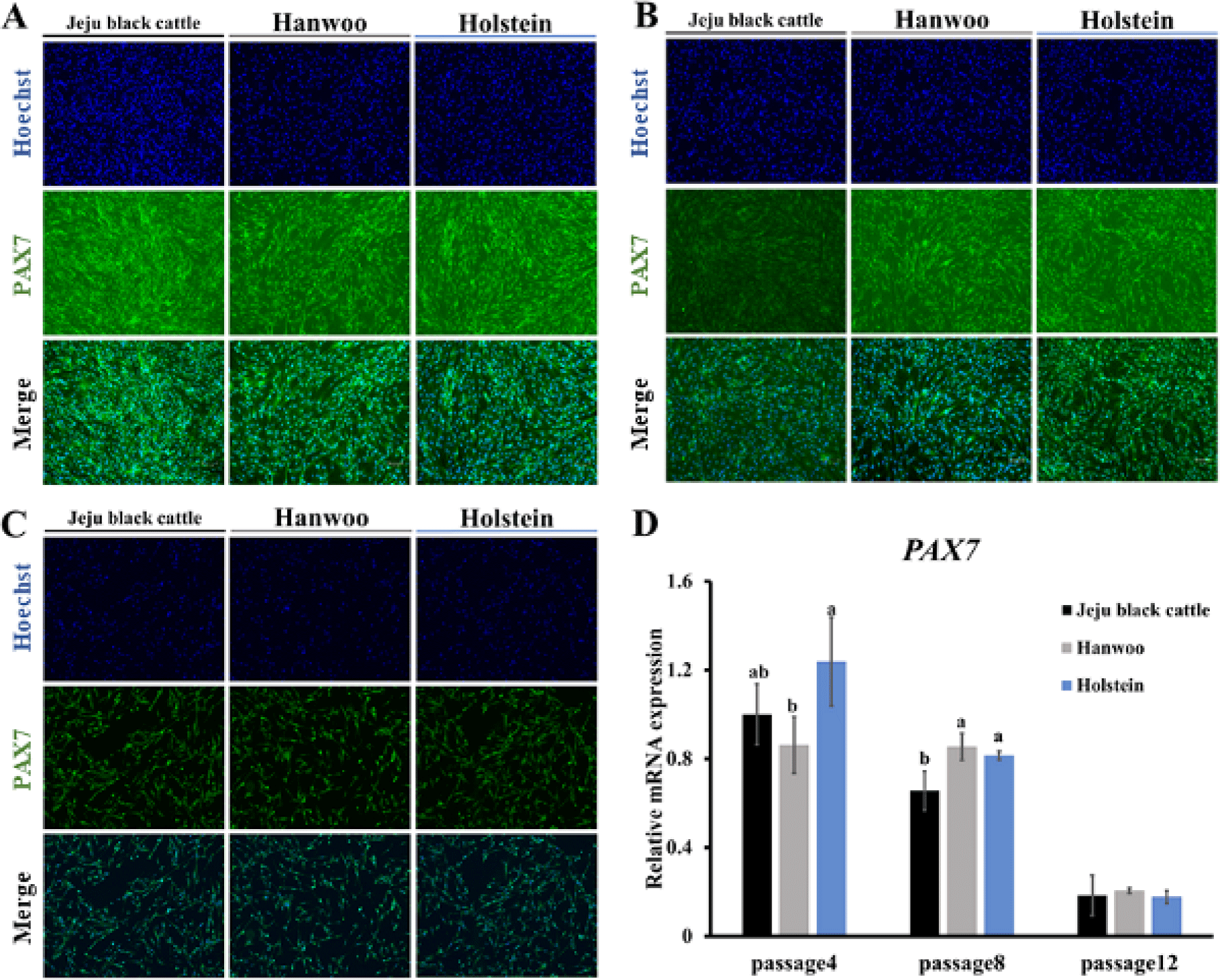
MYOD is a transcription factor that plays an essential role in muscle formation, differentiation, and maintenance during muscle development and regeneration [20]. Myoblast activation is something that MYOD is able to cause. MYOD is one of the muscle control factors that is expressed in dividing myoblasts [21]. The absence of MYOD negatively affects muscle regeneration and delays the transition from proliferation to differentiation of satellite cell-derived myocytes [22]. To confirm the potential for muscle satellite cell proliferation and differentiation into myotubes, MYOD factor RT-qPCR and immunofluorescence staining were conducted. Muscle satellite cells from three different breeds were grown in identical conditions. Experiments were conducted with day 6 muscle satellite cells of proliferation at passages 4, 8, and 12 (Figs. 5A, 5B, and 5C). Overall, MYOD expression tended to decrease during subculture (Fig. 5D). It was judged that as the subculture progressed, the cell proliferation power decreased and the possibility that muscle satellite cells could differentiate into myotubes decreased as the passage progressed. Comparing the expression amount of MYOD among the three varieties, there was no significant difference in MYOD expression among the three breeds at passage 4 (Fig. 5D). However, passages 8 and 12 showed significantly higher MYOD expression levels in Holstein and Hanwoo muscle satellite cells than Jeju black cattle muscle satellite cells (p < 0.05; Fig. 5D). In the case of early passage, all three breeds had similar proliferation and differentiation potential. When the subculture progressed, differentiation capacity of Hanwoo and Holstein muscle satellite cells increased compared to those of Jeju black cattle muscle satellite cells.
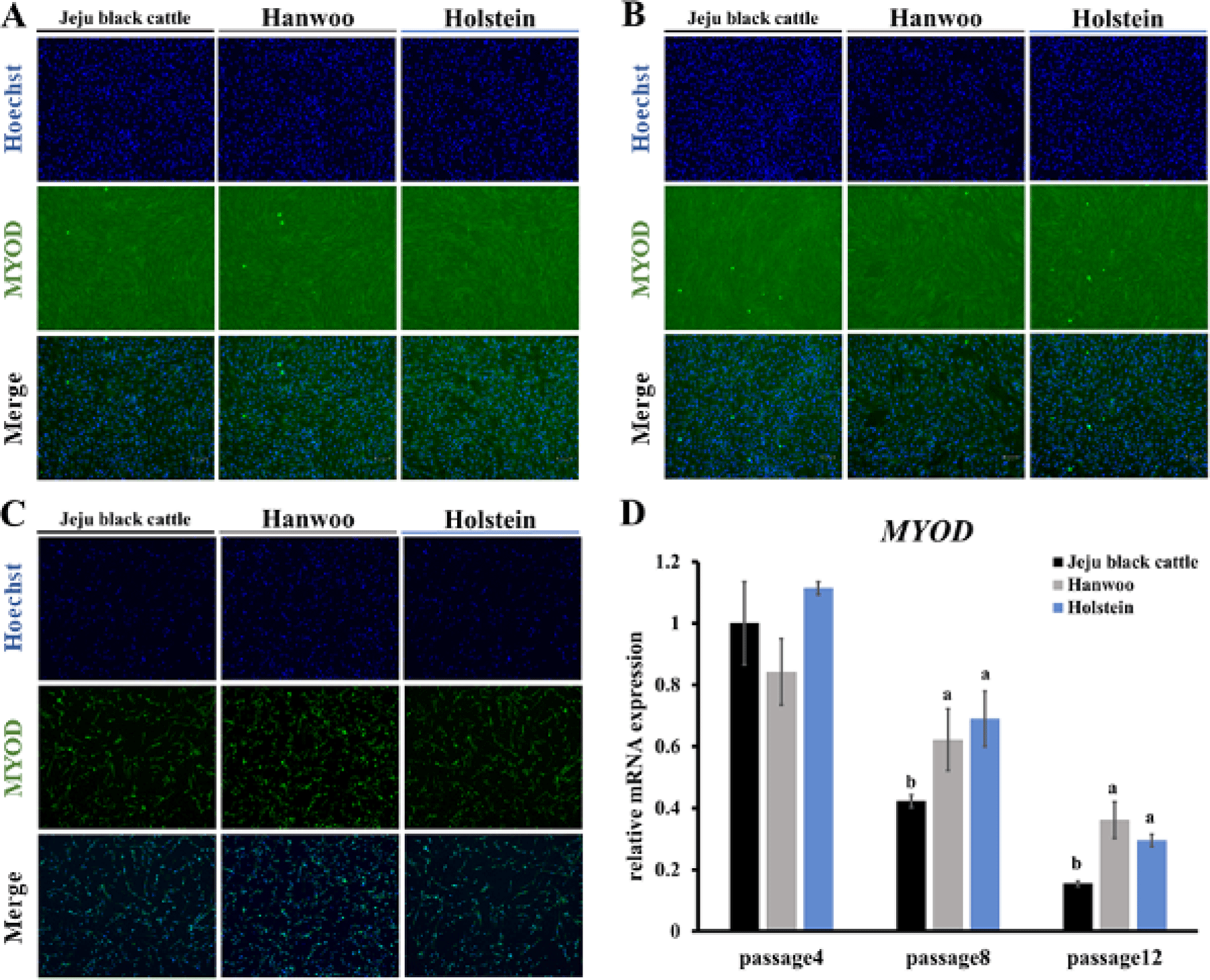
After several proliferation phases, underlying cells begin to differentiate beyond the cell cycle. The next goal is to differentiate them into skeletal muscle cells with the maximum protein production, that is, hypertrophy. A sufficient number of myoblasts obtained through proliferation will return to a stationary satellite cell state, exit the cell cycle, and develop into myotubes through inter-cellular fusion [23]. After inducing muscle satellite cell differentiation of each breed, the tendency of myotube differentiation of three breeds was compared through microscopic observation for 5 days (Figs. 6A, 6B, and 6C). Differentiated muscle satellite cells in passage 4 began to converge on day 1 and completely formed myotubes on day 3 (Fig. 6A). For passage 12 (Fig. 6C), there was little differentiation of myotubes, with the rate of differentiation being slower than those of passage 4 and 8. It was judged that as the subculture progressed, the differentiation speed and ability of muscle satellite cells from the three breeds of cattle decreased.
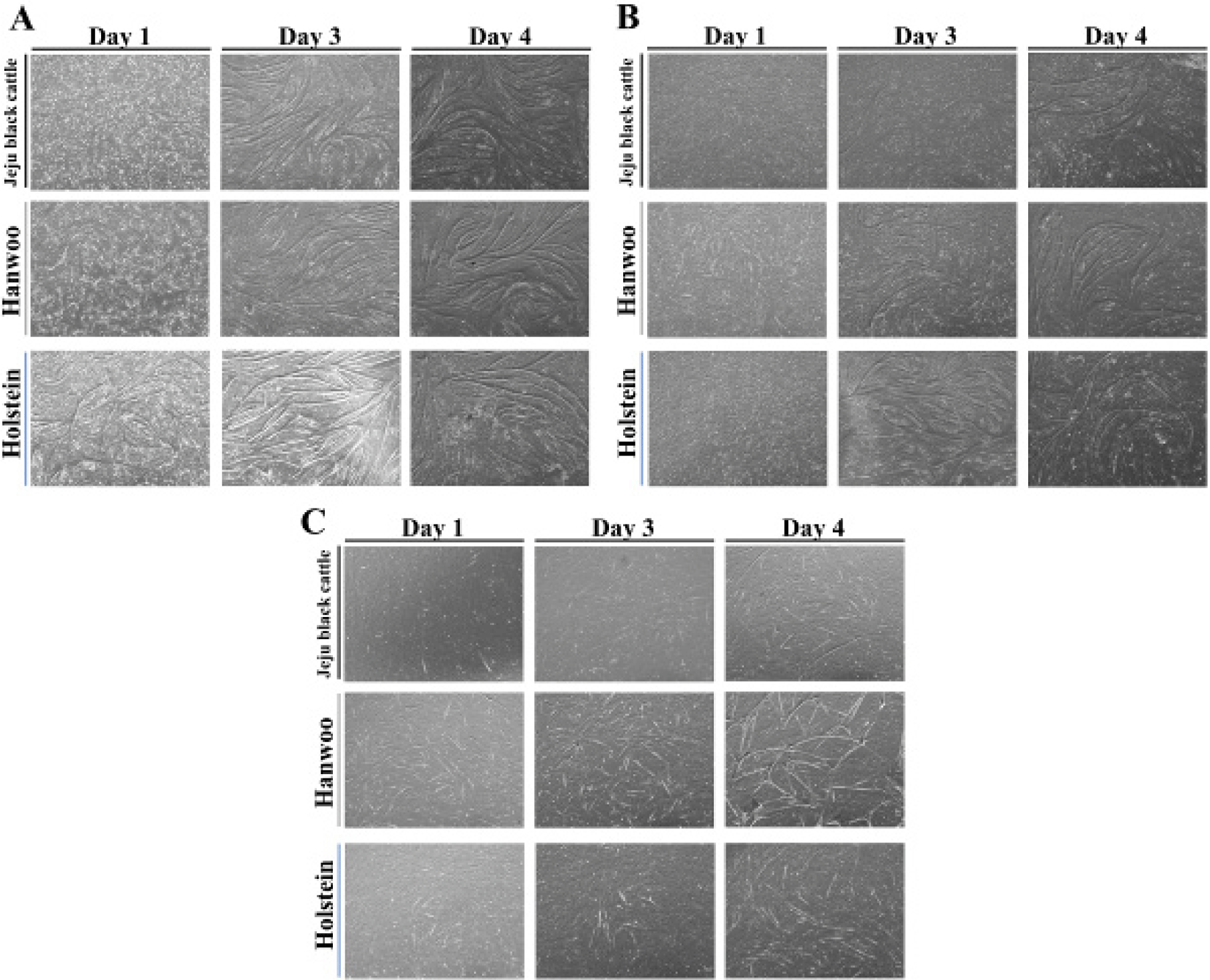
The speed and degree of differentiation of muscle satellite cells in three breeds of cattle were examined. In addition, after muscle satellite cells were differentiated at passages 4, 8, and 12, myosin and nuclear immunofluorescence staining were performed to compare degrees of differentiation of the three kinds of differentiated muscle satellite cells (Fig. 7A). Immunofluorescence staining was performed when three breeds of muscle satellite cells were differentiated. At passage 4, Holstein and Hanwoo breeds’ satellite cells had high degrees of differentiation than Jeju black cattle’s muscle satellite cells based on immunofluorescence image analysis using ImageJ. After calculating widths of myotubes and areas occupied by myotubes, Holstein’s muscle satellite cells showed the highest values (Figs. 7B and 7C). Fusion index values were also significantly higher for Holstein and Hanwoo breeds’ satellite cells (p < 0.05; Fig. 7D). Thus, Holstein’s muscle satellite cells have a higher degree of differentiation than the other two breeds’ satellite cells when all cells are differentiated at passage 4. In comparison to slowly developing cattle, faster maturing cattle (Angus compared to Holstein) typically have greater muscle fibers of all kinds [24]. Also, Experiments by Hegarty et al. [25] reported similar trend that early maturing pigs have larger muscle fibers. Even in salmon (Salmo salar L.), early maturing population have longer and greater fibers than late maturing population [26].
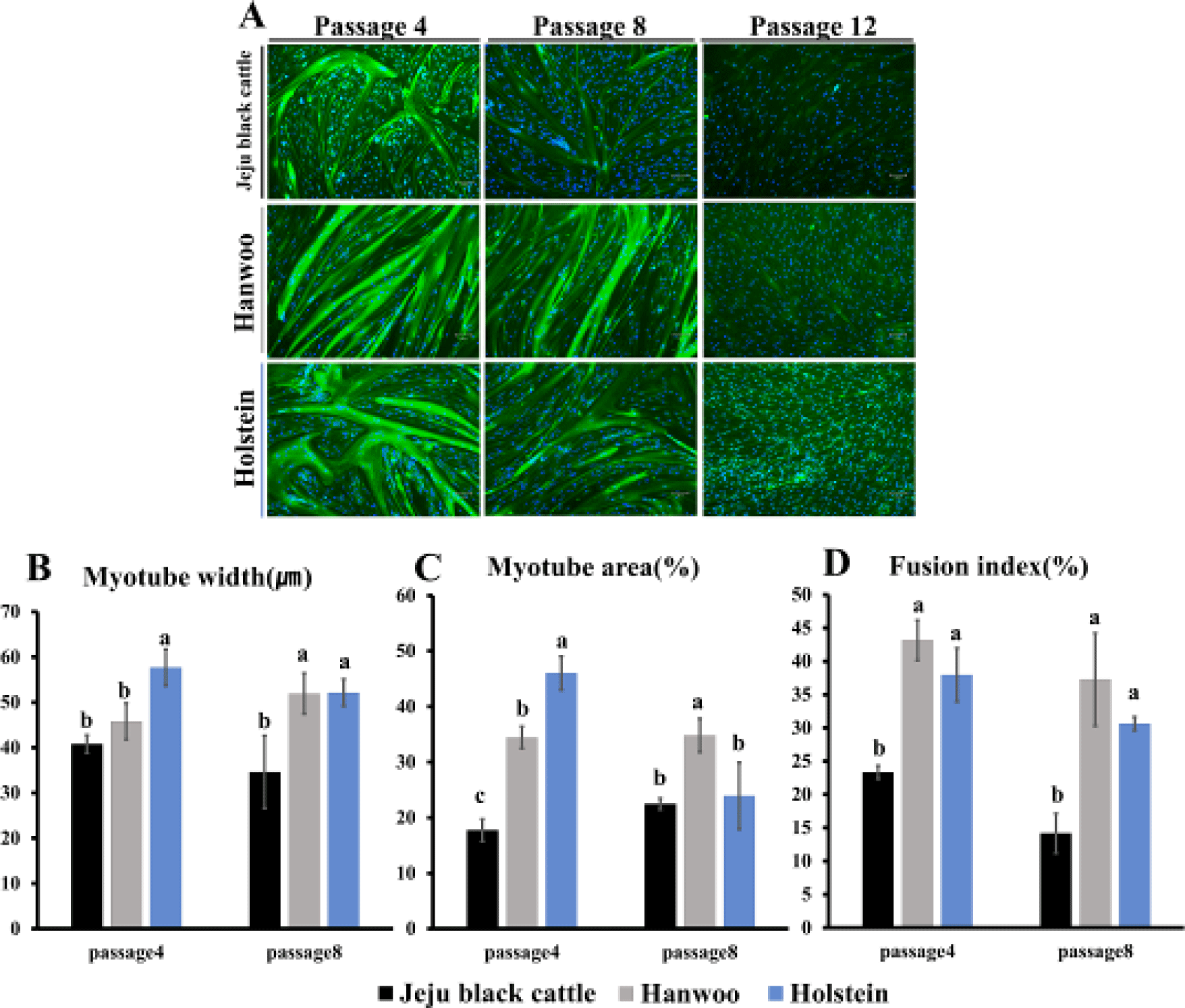
To analyze levels of cattle muscle cell differentiation marker mRNA of three breeds, subculture was performed and quantitative comparison of muscle cell differentiation was performed. Myogenin (MYOG), desmin (DES), myosin heavy chain 4 (MYH4), caveolin 3 (CAV3), insulin like growth factor 1 (IGF1), and troponin T1 (TNNT1) in cells at the time of maximum differentiation during differentiation were analyzed. MYOG, MYH4, and DES are all markers of myocyte differentiation. MYOG expression is crucial for the differentiation of muscle cells [21]. MYOG are known as transcription factors for inducing fusion [27]. MYOG is abundant in myotube and muscle fibers. It helps to determine and maintain of types of skeletal muscle fibers [28]. In passage 4, Holstein muscle cells showed significantly higher MYOG expression than other breeds of muscle cells (p < 0.05). In addition, in passage 8, Holstein showed significantly higher MYOG expression (p < 0.05). It was confirmed that Holstein muscle cells had a higher expression of MYOG, a factor that could control differentiation, than the other two breeds’ muscle cells (Fig. 8A). DES encodes subunit proteins of intermediate filaments of skeletal muscle tissues, smooth muscle tissues, and myocardial tissues. It is an extremely abundant intermediate filament protein that is unique to muscles [29]. It is connected to muscle formation, proliferation, differentiation, and fusion of muscles. It is also in charge of ensuring that muscle cells operate normally [30]. During Myogenesis, DES is expressed in differentiated muscle cells, muscle tubes, and muscle fibers. It is one of the crucial indicator of muscle differentiation [31]. In passage4, Holstein muscle cells showed significantly higher DES mRNA expression than the other two breeds’ muscle cells (p < 0.05). In addition, at passage 8, there was no apparent difference in DES mRNA expression among all breeds of cattle muscle cells (Fig. 8B). The main source of fiber motor protein needed for muscle contraction is MYH protein. The MYH gene produces its encoding [32]. Cells of the skeletal muscle express MYH. Myotube formation can be aided by it. Its expression and more muscle mass are positively associated [33]. Muscle-specific proteins such as DES and MYH4 are important for the contraction function of muscle fibers. MYH4 is a marker that affects muscle mass. It is involved in fusion into myotubes. MYH4 showed significantly higher mRNA expression at passage 4 in Holstein muscle cells than in the other two breeds’ muscle cells (p < 0.05). In addition, in passage 8, Holstein muscle cells showed significantly higher expression of MYH4 mRNA (p < 0.05; Fig. 8C). Skeletal muscles are where CAV3 is expressed most frequently. CAV3 contributes to proper differentiation of myofibrils and homeostasis of myofibrils [34]. CAV3 is also expressed in mature multinucleated myotubes. It is mainly expressed in the final differentiation stage. It plays an essential role in the fusion of mononucleated myoblast cells into multi-nucleated myotubes [35]. CAV3 contributes to differentiation and myofibrils homeostasis. It is a marker of mature multinuclear myotube. CAV3 showed significantly higher expression at passage 4 in Holstein muscle cells than in the other two breeds’ muscle cells (p < 0.05). At passage 8, Holstein muscle cells showed significantly higher expression of CAV3 than the other two breeds’ muscle cells (p < 0.05). Overall, Holstein’s expression was measured to be significantly higher (p < 0.05; Fig. 8D). IGF1 can stimulate MRFs such as MYOD and MYOG [36]. IGF1 is allocated to biological processes such as myoplasia, myofascial proliferation, myofascial differentiation, and myofascial fusion [30]. IGF1 also contributes to the development and maintenance of muscular tissue [37]. IGF1 is temporarily expressed after muscle cell differentiation. It can promote muscle fiber enlargement in a self-secreting manner [38]. Higher IGF1 expression levels may contribute to higher muscle mass growth. IGF1 is a marker that stimulates MRF during differentiation. It is involved in muscle hypertrophy. at passage 8, Holstein’s muscle cells showed significantly higher expression of IGF1 than the other two types of muscle cells (p < 0.05; Fig. 8E). TNNT1 is a gene involved in muscle formation, such as MYOG and CAV3. TNNT1, a skeletal muscle marker, is only observed in differentiated cells. TNNT1 is a subunit of tropomyosin binding. It can combine with tropomyosin to form a troponin-tropomyosin complex, which is crucial for controlling the contraction of striated muscle. TNNT1 is a differentiation-specific marker observed in differentiated myotube. AAt passage 4 and 8, TNNT1 showed significantly higher in Hanwoo and Holstein muscle cells than Jeju black cattle (p < 0.05; Fig. 8F). These results can be associated with the characteristics of cell donor breeds.

Among Holstein, Hanwoo, and Jeju black cattle, Holstein breeds show the largest and highest growth. Satellite cells are deeply related to muscle formation and growth in vivo. It is judged that Holstein muscle satellite cells have better proliferation and differentiation capacity in vitro than muscle satellite cells of Hanwoo and Jeju black cattle due to the genetic factors of the breed.
CONCLUSION
The purpose of this study was to investigate and compare proliferation and differentiation characteristics of satellite cells from three breeds of cattle distributed in Korea. In this study, an experiment was conducted with an emphasis on cattle breeds. However, gender, environmental factors, and conductor age of functional elements are also factors affecting the selection of donors for culture meat production. Although more researchs are needed, this study emphasized the importance of selecting donors. It sought to identify characteristics of donors for differences between breeds. In this case, Holstein can be optimally selected as satellite cell donors for beef culture meat production due to the faster proliferation potential and stronger differentiation capacity of satellite cells than Hanwoo and Jeju black cattle. It will help us select the best donor to produce beef culture meat in Korea. Results of this study provide insight into the choice of donor for cultured meat.