INTRODUCTION
Mycotoxins are toxic secondary metabolites formed by several molds, especially aspergillus, penicillium, and fusarium [1] that could contaminate food and feed at various stages in the animal feed chain. Despite numerous modern and improved management practices implemented in the agricultural and feed manufacturing industries, mycotoxin contamination of animal diets remains a challenge [2]. Pigs are sensitive to mycotoxins ingestion [3]. Furthermore, pig diet formulations are dependent on cereals, a high-risk factor for mycotoxin exposure [4]. The major mycotoxins that contaminate animal feeds include aflatoxins, ochratoxin, zearalenone, fumonisins, trichothecenes, deoxynivalenol (DON), trichothecene-2 toxin, and hydroxytrichothecene-2 toxin [3]. Mycotoxin contamination decreases growth efficiency, lowers feed conversion rate, reproductive rates, and impairs resistance to diseases in domestic animals such as dairy cattle, sheep, swine, and poultry [5,6]. They increase the vulnerability of animals to infectious diseases, decrease vaccine effectiveness, and cause pathological harm to body tissues, kidneys, liver, and the reproductive, nervous, and immune systems [7,8]. Some mycotoxins are carcinogenic, genotoxic, and teratogenic [9]. Consequently, it negatively affects the health of animals. Overall, apart from the negative impacts on production imposed by mycotoxin contamination in swine production, farmers equally incur huge costs to combat the harmful effects caused by mycotoxins in animals. This increases the cost of production hence diminishing pork producers’ profits. This calls for cost-effective strategies to overcome the challenges of mycotoxins in livestock production.
Most studies on mycotoxicosis alleviation have focused on methods of physical and chemical mycotoxin degradation [10] and the use of adsorbents, including aluminosilicates [11] and esterified glucomannan [12]. However, in vivo studies have shown that these approaches are not always effective against chemically diverse mycotoxins. [11]. Currently, research on nutritional therapy to treat mycotoxin-induced effects in swine has focused on dietary supplementation of amino acids (AA) in mycotoxin compromised animals’ diets. AA are nutritional components that have been found to enhance the immune function of animals. Some are essential for regulating major metabolic pathways; hence, they are known as functional AA [13]. This class of AA includes arginine (Arg) and methionine. Previous studies [14,15] conducted on pigs supplemented with higher amounts of Arg or methionine showed an improvement in growth performance and antioxidant status during heat stress. L-Arg is a conditionally essential AA in swine [16]. It stimulates intestinal development and maturation [17], further, it increases phagocytic activity, and accelerates the elimination of endotoxins in the gut and other organs [18]. Furthermore, mucosal microcirculation and absorption are enhanced by Arg [19]. It promotes the expression of anti-oxidative genes [20] through the nitric oxide (NO) synthesis mechanism. Moreover, Arg plays a vital role in swine nutrition regulatory functions, especially in immune response and nutrient metabolism [21]. Therefore, the present study aimed to evaluate the beneficial effects of L-Arg supplementation in growing pigs fed a mycotoxin-contaminated diet on growth performance, immune system, and major body organs
MATERIALS AND METHODS
The experiment was conducted with proper observation of ethical standards and institutional protocols approved by the Kangwon National University Animal Welfare and Use Committee in the Republic (210503-6).
This study was performed in Kangwon National University Animal Farm, Chuncheon, Korea. Seventy-two cross-bred (Landrace × Large white) 21-day-old piglets of mixed sex, 1/2 of the entire males and 1/2 females of initial body weight (BW) 55.2 ± 2.5 kg were randomly allotted to one of the four dietary treatments; NT1.2 (non- toxin basal diet with 1.2% Arg), TX1.2 (mycotoxin-contaminated basal diet with 1.2% Arg), TX1.3 (mycotoxin-contaminated basal diet with 1.3% Arg), and TX1.4 (mycotoxin-contaminated basal diet with 1.4% Arg). The contaminated feed was mildewed under ambient conditions (temperature, 23°C–28°C; humidity, 68%–85%), as described by Liu et al. [11]. Liquid chromatography (Beijing Taileqi, Beijing, China) was used to detect the mycotoxins (Table 1). Basal diets were composed of a corn-soybean meal formulated as recommended by the NRC [22] to meet or exceed the nutritional requirements of growing pigs (Table 2). Arg was supplemented at the rate of 1.2%, 1.3%, and 1.4% per kg of the basal diet throughout the 20 days experimental period. Every treatment had three replicate pens composed of six pigs per pen (3 male; 3 female) group-housed. The experimental room contained 12 pens (2.28 × 1.85 = 4.2 m2) per pen with slatted floors. The room was equipped with automated fan-assisted central heating, forced ventilation and artificial lighting to ensure a minimum of 12 h of light/day. Every pen was provided with; one hopper-type feeder with two feeding points and a nipple drinker. Throughout the experiment, piglets had unlimited access to water and feed. Routine analysis of the diet and drinking water was performed for any contaminant that could affect the integrity of the study. On day 20 of the study, two pigs from each treatment group were euthanized by approved anesthesia and exsanguination, and tissue samples were harvested.
1) Supplied per kilogram of diet: 16,000 IU vitamin A, 3,000 IU vitamin D3, 5.0 mg vitamin B1, 20 mg vitamin B2, 4 mg vitamin B6, 0.08 mg vitamin B12, 40 IU vitamin E, 5.0 mg vitamin K3, 75 mg niacin, 40 mg pantothenic acid, 0.15 mg biotin, 0.65 mg folic acid.
Blood samples were collected from two pigs per group from the jugular vein and centrifuged at 3,000 rpm for 10 min at 4°C. The serum was transferred to Eppendorf tubes and stored at −20°C until analysis. Interleukin (IL)-4, tumor necrosis factor α (TNF-α), IL-6, toll-like receptor-4 (TLR-4), and interferon-gamma (IFNγ) levels were measured using ELISA test kits (Beijing Laboratory Biotech, Beijing, China).
The kidneys, spleen, heart, and liver were removed and weighed, and the organ weight and total BW percentage were recorded.
Gut samples were collected from the duodenum, jejunum, and ileum, frozen in liquid nitrogen at −80°C, and stored for analysis of intestinal histomorphology. The duodenal, jejunal, and ileal samples were cut into 5 cm lengths and fixed for 24 h in 10% neutral buffered formalin, transferred to a solution of 70% ethanol, embedded in wax, sectioned, and stained with eosin and hematoxylin. Slices were mounted on slides for analysis as described by Hosseindoust et al. [23]. Intestinal morphology was evaluated by measuring the height and crypt depths (CD) of the most prominent villi from each of the three sections using an Olympus Vanox-S Microscope (Olympus Corporation, Lake Success, NY, USA) and analyzed using Spot basic imaging software (Diagnostic Instruments, Sterling Heights, MI, USA).
All the experimental pigs were weighed individually on the first and last day of the experiment. Feed consumption was also recorded to calculate the average daily feed intake (ADFI), average daily gain (ADG), and gain: feed ratio.
Complete RNA was extracted from liquid nitrogen-pulverized tissues using TRIzol reagent (Invitrogen, Waltham, MA, USA) and treated with DNase I (Invitrogen) as directed by the manufacturer and described by Hosseindoust et al. [24]. After staining with 10 g/mL ethidium bromide, RNA purity was evaluated using 1 percent agarose gel electrophoresis. The optical density (OD)260 : OD280 ratio of the RNA was between 1.8 and 2.0. First-strand cDNA was synthesized using oligo (dT)20 and Superscript II reverse transcriptase (Invitrogen).
Primer 5.0 was used to design primers based on the pig’s cDNA sequence (Table 3). To standardize the target gene transcript levels, -actin was used as the housekeeping gene. SYBR Green PCR Mix, comprising MgCl2, dNTP, and Hotstar Taq polymerase, was used for real-time PCR. cDNA template (2 µL) was added to a 25 µL mixture including 12.5 µL of SYBR Green solution and 1 mol/L forward and reverse primers. The following protocol was used: (a) pre-denaturation (10 s at 95°C); (b) amplification and quantification, repeated 40 times (5 s at 95°C, 20 s at 60°C); and (c) melting curve (60°C–99°C with a heating rate of 0.1°C s−1 and fluorescence measurement).
All the results were expressed as mean ± standards error of the mean, statistical analyses were done using the GLM procedure of SAS (SAS Institute, Cary, NC, USA) based on a completely randomized design. Significant differences of (p < 0.05) among the treatment were considered statistically significant differences using Turkey’s Honestly Significant Difference procedure. Pig was considered as an experimental unit for all parameters.
RESULTS
Growth performance, as measured and evaluated by BW, ADG, ADFI, and feed conversion efficiency, is presented in Table 4. Overall, final BW (p < 0.05), ADG (p < 0.05), and gain: feed ratio (p < 0.05) was significantly higher in the NT1.2 treatment group as compared to all three toxin treatment groups (TX1.2, TX1.3, and TX1.4). No significant differences were observed in the ADFI (p > 0.05) among the groups.
There was no significant difference (p > 0.05) in the relative weights of the kidney, heart, and spleen among the groups. However, the relative weight of the liver was significantly higher (p < 0.05) in the TX1.2 group compared with the NT1.2 and both the TX1.3 and TX1.4 groups, with the NT1.2 group having the lowest liver weight (Table 5).
The villous height (VH) in the duodenal section of the gut in the NT1.2 group was statistically higher, although not significant (p = 0.074; Table 6). No significant differences (p > 0.05) were observed in the jejunum and ileum of the intestine in terms of VH, CD, and VH:CD ratio among the groups.
There were no significant differences (p > 0.05) in the liver IL-4, IL-6, IFNY, and TLR-4 levels. The mRNA level of TNFα in the liver tissue was significantly down-regulated in the non-toxin, TX1.3, and TX1.4 treatment groups as compared with the TX1.2 at (p < 0.05; Figs. 1A–1E). In contrast, significant differences were observed in mRNA level changes in muscle IL-6 (p < 0.05) and TNFα (p < 0.05) between the NT1.2 and the TX1.2 treatment groups, although not different from TX1.3 and TX1.4. The mRNA levels of the other cytokines and TLR-4 were not significantly different (p > 0.05) among the groups (Figs. 2A–2E). There was no statistical difference (p > 0.05) in the changes in the mRNA levels of all cytokines and TLR-4 in the jejunum among the groups (Figs. 3A –3E).
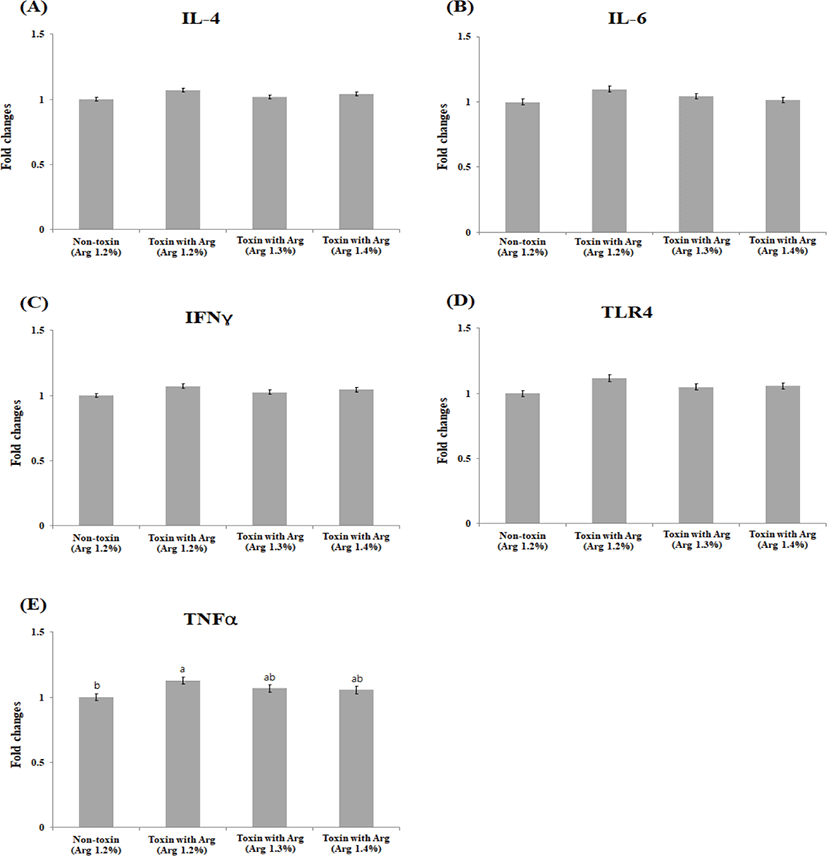
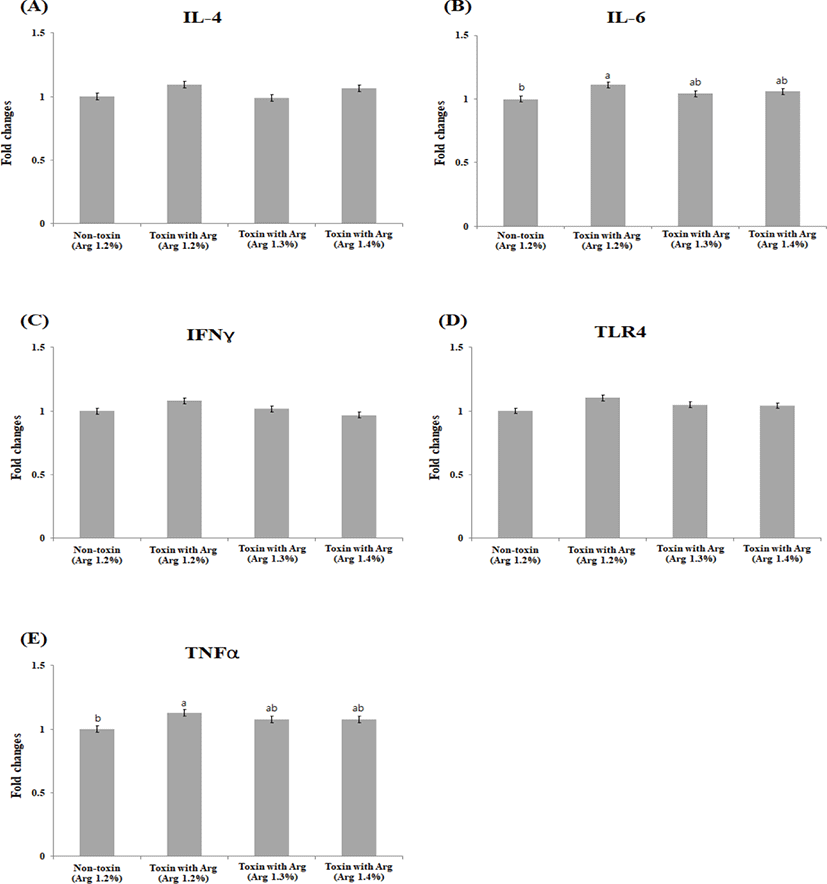
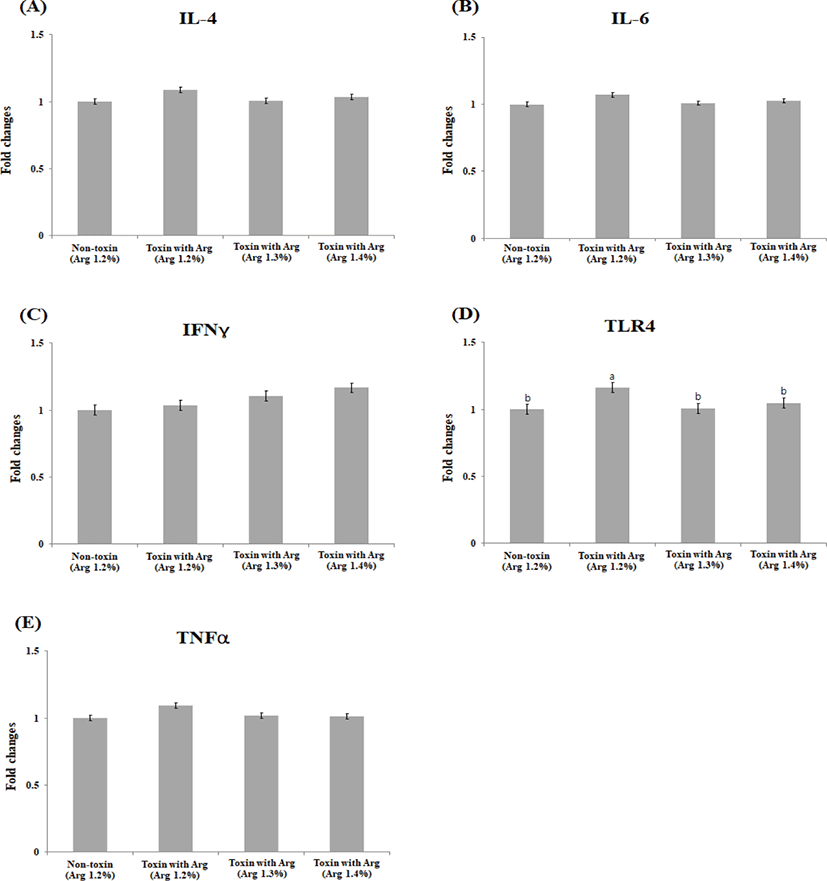
DISCUSSION
L-Arg is an essential AA that is a precursor for synthesizing urea, NO, polyamines, creatine, and proteins and modulates the intestinal gene expression of cationic AA transporters and antioxidants [16,20]. Arg is vital for growth performance improvement and the morphological development of the small intestine in growing pigs fed on a mold-contaminated diet [15,16]. Results from a previous study showed that the consumption of mycotoxin-contaminated feeds led to decreased ADG and ADFI, thereby lowering growth performance in pigs [13]. In this study, mycotoxin contamination negatively affected BW, ADG, and feed conversion efficiency. These results are consistent with a previous study that reported a significant decrease in pig performance by the ingestion of DON-contaminated feeds [24]. Previous studies have shown great damage to intestinal function through an impaired gut barrier, damage to the intestinal structure, and reduced immunity [25,26]. Disruption of intestinal function interferes with the normal absorption of the end products of digestion into the bloodstream; hence, fewer nutrients are available for growth [27]. In our study, supplementation with various levels of Arg did not mitigate growth suppression caused by mycotoxins. Similar to our results, a previous study on supplementation with glutamine and Arg failed to overcome growth suppression induced by mycotoxins in grower pigs [13]. However, in contrast to our findings, previous studies have shown positive results in terms of a higher growth rate on supplementation with peptides and AA preparations in alleviating mycotoxicosis in pigs and mice [28,29]. This may have been due to the combined effects of various peptides, each with an additive effect contrary to our study with only Arg as the only AA supplemented hence lacking the complementary advantage when many peptides and AA are supplemented.
Because of its detoxification function, the liver is a target organ for mycotoxins, leading to edema and liver enlargement [3]. Similarly, Yarru et al. [30] reported an increase in liver weight and accumulation of fat in the liver, resulting in disruption of hepatic functions. In our current study, the weight of the liver was significantly higher in the TX1.2 group compared to that of the NT1.2, TX1.3, and TX1.4 groups. The lower liver weights in the TX1.3 and TX1.4 groups could have resulted from attenuation of the toxic effect of mycotoxins with higher levels of Arg supplementation, which could have improved the hepatic function of the liver, preventing edema. These findings imply that Arg protect the immune system and minimize liver harm in pigs exposed to mycotoxin. We hypothesized that Arg could increase NO production in the small intestine and other tissues, which is important for regulating the antioxidant defense system and scavenging excess reactive oxygen species induced by moldy feeds, thus improving the balance between reactive oxygen species production (e.g., superoxide anion, hydrogen peroxide, and hydroxyl radical) and biological defense against their toxicity. Similar to our results, a previous study reported a 10.7% increase in liver weight in a DON-stressed toxin control group compared to a non-toxin control in growing pigs [13]. Furthermore, Trenholm et al. [31] reported an increase in kidney and liver weights in growing pigs fed diets with higher DON concentrations and prolonged ingestion of feed contaminated with mycotoxins. However, a previous study [32] found no significant weight change in the liver, spleen, or kidneys of growing pigs fed a diet contaminated with aflatoxins and DON. In our current study, there was no significant difference in the relative weights of the kidney, heart, and the spleen among the treatments, we attributed this to be due to increased protein synthesis as a result of Arg supplementation and organ expansion induced by severe mycotoxin systemic toxicity interacting competitively to influence relative organ size
IL-6, IFNY, and TNF-α are pro-inflammatory cytokines associated with porcine immune status. TNF-α is involved in systemic inflammation [33]. It is one of the first response cytokines produced following mycotoxin exposure, especially ochratoxin [34], when the immune system is stimulated. Therefore, its gene expression is up- regulated in the liver and muscle following mycotoxin toxicity. The liver is the first organ to offer a protective barrier against mycotoxin absorption in the gut because it prevents the systemic distribution of toxins throughout the body [35]. In our current study, the expression of both IL-6 and TNF-α were significantly lower in the NT1.2, TX1.3, and TX1.4 treatments compared to the TX1.2 treatment. This may be due to the protective effects of the increased Arg level on the immune system, thereby down-regulating TNF-α and IL-6 production and reducing inflammatory responses in both the muscle and liver. We hypothesized that this may have been due to NO production as a result of Arg metabolism. NO is a vital signaling molecule that plays a role in, vascular homeostasis, neurotransmission, immunological modulation, and host defense [18,21,36] hence counteracting the suppressive effects and abnormal regulation of metabolic pathways and immunological responses [37]. Similar to our findings, Zhou et al. [38] reported an increased expression of TNF-α and IL-2 mRNA in pigs fed a diet contaminated with DON. TLR-4 is a pattern-recognition receptor subtype expressed at high levels along the intestinal tract. It acts as the first immune barrier in the gastrointestinal innate immune system. TLR-4 plays a key role in translating and transcription of inflammatory mediators [39]. Our research found that the level of TLR-4 expression was significantly higher in the TX1.2 treatment compared to that of the NT1.2 group. This could result from the toxicity eliciting the up-regulation in the level of TLR-4. Notably, the expression level was not significantly different in the TX1.3 and TX1.4 groups compared to that of both the negative and positive controls, which could indicate the ability of higher levels of Arg to counteract the effects of mycotoxin toxicity, hence modulating immune responses. As a result, we hypothesized that exogenous Arg would improve animals’ metabolism engaged in mitogen-activated protein kinase and nuclear factor-kappaB pathways, reducing the impairment generated by mycotoxins stress and improving immune important cytokines such as IL-4 which is an anti-inflammatory cytokine even though in this study no significant difference was observed across the groups in all the tissues under study. This may have been due to the concentration of the mycotoxins used and the relatively shorter period (20 days) which the animals were exposed to the treatment diets.
In conclusion, high dietary Arg supplementation in mycotoxin-contaminated growing pigs significantly reduced morphological liver injury induced by toxicity from ingesting mycotoxin-contaminated feed. Furthermore, higher levels of Arg significantly down-regulated the pro-inflammatory cytokine TNF-α, protecting the immune system and even the liver. This improved the health of mycotoxin-compromised animals.