INTRODUCTION
Intramuscular fat (marbling) is the most important factor determining the beef quality and directly affects profitability. Various methods have been investigated, and substantial efforts have been made to efficiently increase intramuscular adipogenesis [1-3]. Most of the previous studies have focused on the fattening period. However, maternal nutrition during gestation has recently been shown to affect offspring growth and intramuscular fat [4,5]. Consequently, interest in fetal programming has increased. It is important to understand fetal growth patterns throughout gestation to apply fetal programming effectively. Fetal development in ruminants shows patterns of organ formation in early gestation, myogenesis in mid-gestation, and adipogenesis in late gestation [6]. However, few studies have assessed gene expression and the development of intramuscular adipocytes in fetuses during gestation.
Controlling the nutritional level of pregnant cows is one of the most useful strategies to maximize offspring performance. The level of nutrition consumed by the mother during gestation plays an important role in fetal growth and development [7]. It also affects the acquired growth and physiological function of offspring, which can change the productivity and quality of livestock [8]. Although many nutrients are present in the feed, a single nutrient can affect fetal programming [9]. Proteins are essential nutrients for the production of enzymes and hormones, as well as for cell proliferation and differentiation. Recent studies have shown that high-protein grass or concentrate intake in pregnant cows improves fat storage in the offspring [9,10]. These results suggest that the protein may positively affect intramuscular adipocyte differentiation in the fetus. However, excess protein during gestation period induces oxidative stress in the fetus [11] and reduces insulin-like growth factor-I levels in the maternal blood, affecting the distribution of nutrients between the dam and fetus [12]. One important commonality is that excessive maternal nutrient supplementation reduces the birth weight of offspring and impedes their growth [11,13,14]. To avoid this problem, it was necessary to set an appropriate increment range.
We hypothesized that potential intramuscular fat can be formed during the fetal period and that increase of crude protein (CP) intake during pregnancy could increase economic profitability through improved growth and meat quality (marbling) of male offspring without negative impact. Therefore, the first objective of this study was to investigate the growth, intramuscular adipogenesis, and expression of major genes in fetuses during gestation. The second objective was to investigate the effect of increase in CP intake during pregnancy on body weight (BW) and subsequent reproduction in the mother and evaluate the productivity and economics of male offspring born from them.
MATERIALS AND METHODS
Eighty six multiparous Hanwoo cows (average weight 551.5 ± 51.3 kg, age 5.29 ± 0.61 y, parity 3.52 ± 0.23) were used in the present study. Hanwoo cows underwent artificial insemination (AI) after estrus synchronization, and pregnancy was detected using rectal ultrasound 60 days after AI. Eighteen pregnant cows were randomly selected to investigate intramuscular fat formation in fetal. Cows were allocated 6 per group and housed 3 per pen (5 × 10 m), and were fed according to the standard nutrition requirement until slaughter at 90, 180, and 270 d of gestation. To determine the effect on increased CP intake during gestation, 68 pregnant cows were randomly assigned into 2 groups with different feeding levels. The control group (CON) was assigned 34 pregnant cows and provided standard protein requirements [15] (Concentrate Ⅰ: 3.54 kg + rice straw: 4.75 kg). The remaining 34 pregnant cows were assigned to the treatment group (TRT), and high-protein concentrate (II) was provided at a ratio of 3:1 to normally concentrate (Concentrate Ⅰ: 2.66 kg + concentrate Ⅱ: 0.88 kg + rice straw: 4.75 kg). Cows were maintained 4 per pen (5 × 10 m), and concentrate and rice straw were provided twice a day. They were moved to the delivery room 2 months before delivery and managed individually. The ingredients and chemical composition of the experimental diets are listed in Table 1.
1) Vitamin premix provided the following quantities of vitamins per kilogram of the diet: vitamin A, 10,000 IU; vitamin D3, 1,500 IU; vitamin E, 25 IU.
Only male offspring were used to evaluate the fetal programming effect on increased CP intake during pregnancy. After birth, male calves were wiped with a clean towel, and their belly buttons disinfected. The calves were kept in the delivery room with the mother until weaning, and a small amount of starter and grass was provided. Male calves were weaned at 90 d of age and divided into 2 groups according to the protein levels of the pregnant cows: CON male offspring (CON-O) and TRT male offspring (TRT-O). Male calves were allocated 4 or 5 per pen (5 × 10 m) with sawdust to a thickness of approximately 20 cm and surgically castrated at 6 months of age.
The feeding method was divided into growing period and fattening period. Timothy hay was fed in the growing period and rice straw was used in the fattening period. The timothy hay was started with 1.0 kg at birth and increased by 1 kg every month to 4 kg at 7 months of age, and was fixed at 4.5 kg for 8 to 13 months. From 14 months rice straw was reduced by 0.5 kg every 2 months from 3 kg, and was fixed at 1 kg from 22 months of age until slaughter.
Concentrate feed was started to provided 1.5 kg at birth, and the amounts was increased by 0.5 kg every month to 6.5 kg at 13 months of age. At the fattening period, concentrate feed was provided 7.0 kg at 14 months of age, and the amounts was increased by 0.5 kg per months up to a maximum of 10 kg, and fixed at 9 kg from 4 months before slaughter. Feed was provided twice daily, and water and mineral blocks were freely available. The ingredients and chemical compositions of the experimental diets are listed in Table 2.
1) Vitamin premix provided the following quantities of vitamins per kilogram of the diet: vitamin A, 10,000 IU; vitamin D3, 1,500 IU; vitamin E, 25 IU.
Pregnant cows were slaughtered at different gestation periods (90, 180, and 270 days). Immediately after slaughter, the fetus was removed from the uterus. The weight and length of the fetuses were measured using an electronic scale and tape measure. The skin and subcutaneous tissues of the fetal longissimus dorsi (LD) and semimembranosus (SM) were removed, and pure skeletal muscle samples were collected. Skeletal muscle samples were divided into two sterile tubes: one was frozen in liquid nitrogen until messenger ribonucleic acid (mRNA) analysis, and the other was fixed with 10% neutral buffered formalin for use in histological analysis.
The LD and SM samples were trimmed so that the cross-section could be inspected, and washed in running water for 4 h. For dehydration of the tissue sample, 10%, 20%, and 30% sucrose solutions were substituted, and then the substituted tissue was put into the optimal cutting temperature (OCT) compound, and the tissue was quickly frozen and embedded. After freezing, each sample was sliced into 10 µm slides using a freezing microtome, and the sliced tissues were mounted on silane-coated glass slides. Tissue slides were washed with phosphate buffer saline (PBS). Five mL of PBS with 0.1% Triton X-100 (Sigma-Aldrich, St. Louis, MO, USA) solution was added to the slide, and incubated at 20°C for 30 min. Tissue slides were washed and blocked with 10% bovine serum albumin (Sigma-Aldrich) and 22.52 mg/mL glycine in PBS + 0.1% Tween 20 (PBST). After blocking, the samples were incubated with primary antibodies against myosin light chain 2 (Abcam, Cambridge, UK) and body-fit solution (4, 4 - Difluoro - 1,3,5,7,8 - Pentamethyl - 4 - Bora - 3a, 4a - Diaza - s - Indacene) for 1 h in the dark environment at 20°C. After washing with high-salt PBS, the samples were incubated with a secondary anti-body solution. Finally, the slides were coated with glycerin, and the slides were covered with a cover glass and observed under an optical microscope (ECLIPSE Ti-U, Nikon, Tokyo, Japan).
2 g Sample and 1ml TRIzol (Ambion, Carlsbad, CA, USA) were put into a screw tube and homogenized with a homogenizer (TissueLyser 2, Qiagen, Hilden, Germany). 100 uL of chloroform was added to the screw tube and vortexed, and centrifuge at 17,000×g for 15 min (4°C). Supernatant 1ml was transferred to a new microtube, and isopropyl alcohol was added, followed by mixing. After centrifuging the microtube at 17,000×g for 15 min (4°C), the supernatant was removed. Again, after adding 1 mL of 75% ethanol and centrifugation at 10,600×g for 5 min (4°C), the supernatant was removed. RNA was dissolved by adding in diethyl pyrocarbonate to the microtube, and synthesized using a cDNA synthesis kit (ReverTra Ace® qPCR RT Master Mix with gDNA Remover, TOYOBO, Osaka, Japan).
The gene expression level was analyzed using performed using a 7500 Fast Real-Time PCR System (Applied Biosystems, Foster City, CA, USA). The myogenic markers used were myogenin (MyoG), myosin heavy chain 1 (MYH 1), and myosin heavy chain 2A (MYH 2A), and the adipogenic markers used were CCAAT/enhancer binding protein β (C/EBPβ), peroxisome proliferator activated receptor γ (PPARγ), and stearoyl-CoA desaturase (SCD). The primer sets used are listed in Table 3. The reaction mix was 5.5 μL of distilled water, 2 μL of cDNA, 1 μL each of forward and reverse primers, 0.5 μL of the probe, and 10 μL of the master mix. The reaction conditions were as follows: 40 cycles of 2 min at 50°C, 10 min at 95°C, 15 s at 95°C, and 1 min at 60°C. The housekeeping gene 40S ribosomal protein S9 (RPS9) served as an internal control to normalize the relative mRNA expression.
The BW of the animals was measured using a cattle weight bridge installed in the barn. The average daily gain (ADG) was calculated based on the BW difference and the number of days of feeding. Dry matter intake (DMI) was calculated by measuring the quantity of residual feed per pen before feeding in the morning, and the feed conversion ratio (FCR) was calculated based on DMI and ADG values.
Calving cows were subjected to AI from the second estrus (approximately 21 d after the first estrus) and service per conception (SPC), defined as the number of service inseminations performed to produce a conception. The conception rate was calculated as the proportion of pregnancies after the first AI.
Male offspring were slaughtered in a slaughterhouse at 30 months. After the carcass was chilled in a refrigerator at 4°C for 24 h, carcass weight was measured. Next, the left side of each carcass was cut between the thirteenth rib and the first lumbar vertebra to determine meat yield and quality. The carcasses were evaluated according to the Korean carcass grading system [16]. Yield grades were evaluated as A, B, and C, and quality grade were evaluated as 1++, 1+, 1, 2, and 3. The auction price was determined as the winning bid price according to yield and quality grades.
A partial budget analysis was conducted to evaluate the economic ramifications. Gross receipts were determined based on the selling price per carcass and product of the male offspring. The operating costs were calculated by adding the feed costs (including commercial concentrate and roughage costs) and other costs (water, power, fuel, veterinary and medicine, automobile, farm implementation, farm building and facilities, miscellaneous materials, interest on borrowed capital, land rent, labor, excretion disposal, and production management costs), as provided by the Livestock Production Cost Survey [17]. Net income was calculated as gross receipts minus the operating costs.
All data were analyzed using SAS package 9.1 software program (SAS Institute, Cary, NC, USA). One-way ANOVA was used for weight (kg), length (cm), intramuscular adipocyte area (%), and gene expression (arbitrary units) in the fetus by the day of pregnancy (90 d, 180 d and 270 d). If there was a significant difference between treatment groups, further analysis was performed by Tukey’s post hoc test. Data from the mothers (CON or TRT) and male offspring (CON-O or TRT-O) based on increased CP intake were analyzed using t-tests. Data are presented as the mean and SEM. Differences between treatments were considered significant at p < 0.05.
RESULTS
Changes in fetal BW and length throughout the pregnancy period of Hanwoo cattle are shown in Table 4. Fetal BW continually increased with the progression of the pregnancy period (p < 0.01); in particular, it increased rapidly between 180 and 270 d of gestation. Fetal length increased by more than 3 times to 84.0 cm at 270 d compared to 25.5 cm at 90 d (p < 0.01).
Item | Days of gestation | p-value | ||
---|---|---|---|---|
90 | 180 | 270 | ||
Body weight (kg) | 0.42 ± 0.04c | 4.6 ± 0.84b | 19.9 ± 1.43a | 0.001 |
Body length (cm) | 25.5 ± 0.95c | 51.7 ± 2.64b | 84.0± 1.35a | 0.001 |
The results of the immunofluorescence staining and adipocyte area in the fetal skeletal muscle throughout the pregnancy period of Hanwoo cattle are shown in Fig. 1. Intramuscular adipocytes were observed around the blood vessels after 180 d of fetal growth, and the size of the muscle fibers increased continuously. The adipocyte area increased at 270 d compared to that at 180 d in both LD and SM. In addition, the adipocyte area was similar between the LD and SM muscles at 180 d but was higher in the LD muscle than in the SM at 270 d.
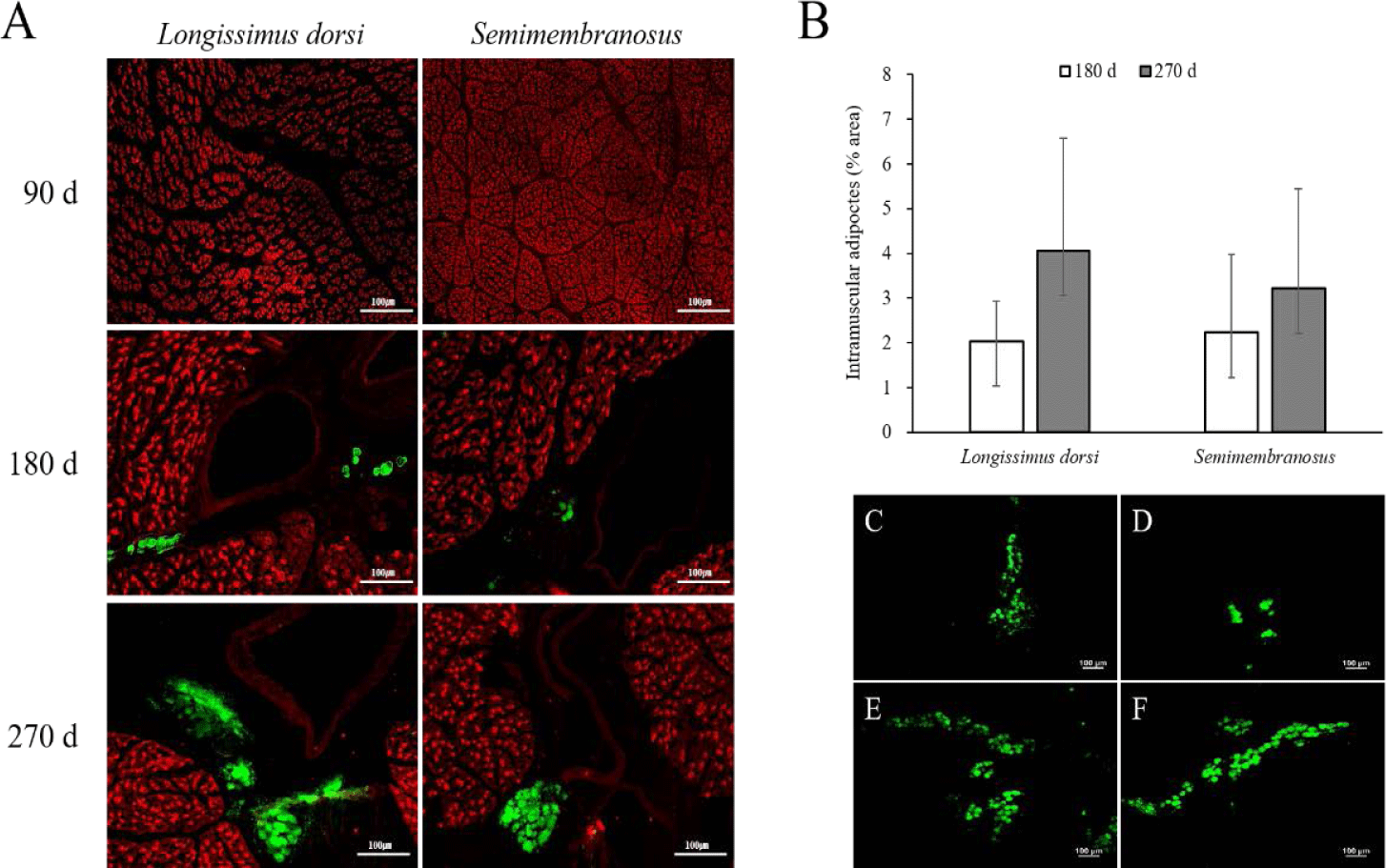
Changes in the mRNA expression of genes related to adipogenesis and myogenesis in fetal skeletal muscle throughout the pregnancy period of Hanwoo cattle are shown in Fig. 2. The mRNA expression of MyoG in LD muscle was significantly decreased at 270 d compared to 180 d of gestation (p < 0.05). MYH1 expression was not affected during gestation, but MYH2A expression was significantly increased at 180 d of gestation (p < 0.05). The mRNA expression of MyoG in SM muscle did not change throughout the pregnancy period, but MYH1 expression was significantly higher at 270 d of gestation (p < 0.05), and MYH2A expression increased throughout the pregnancy period, but not significantly different. The mRNA expression of SCD was greater at 180 d than at 90 and 270 d in LD muscle (p < 0.05). Similarly, the mRNA expression of PPARγ was also the highest at 180 d (p < 0.05). C/EBPβ mRNA expression increased significantly at 180 and 270 d from 90 d of gestation (p < 0.05). No differences were observed in the mRNA expression of SCD and PPARγ in SM muscle, but the mRNA expression of C/EBPβ was affected by the pregnancy period (p < 0.05).
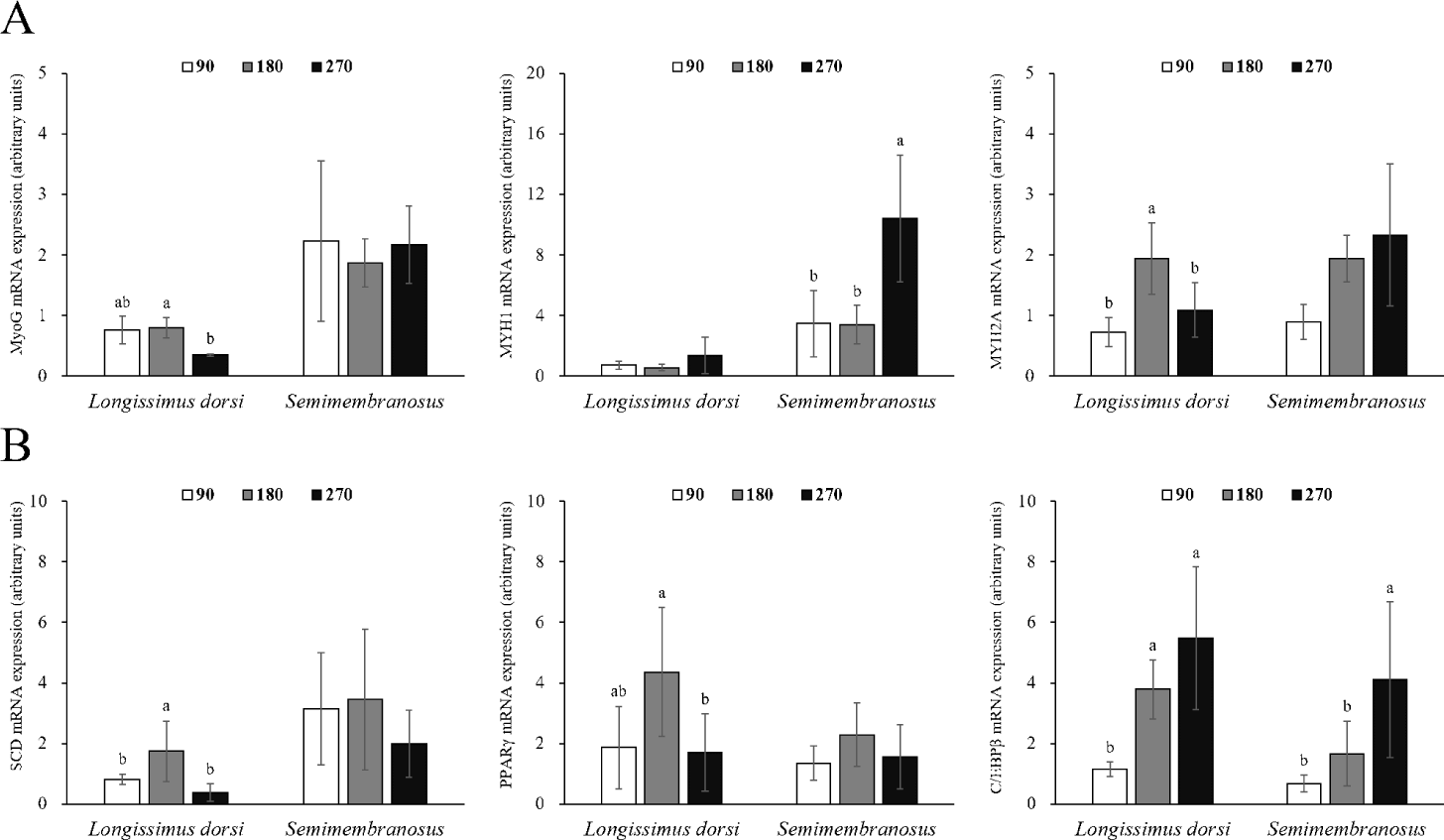
The effects of increased CP intake during pregnancy on growth performance, birth BW, and subsequent reproduction in Hanwoo cattle are shown in Table 5. Protein supplementation did not affect BW, ADG, and DMI of Hanwoo cows during the pregnancy period. The birth BW of female calves was higher in the TRT group than in the CON group (p < 0.01). Similarly, the birth BW of male offspring born in TRT was higher than of those born in CON (p < 0.01). SPC and conception rates were similar between the treatments.
The effects of increased CP intake during pregnancy on the growth performance of the male offspring of Hanwoo cattle are shown in Table 6. The initial BW during the growing period was higher in TRT-O than in CON-O, and the final BW during the growing period was higher in TRT-O than in CON-O (p < 0.04). However, there were no differences in the ADG, DMI, and FCR. The finishing period was, on average, 91.1 kg higher in TRT-O compared to CON-O (p < 0.01). ADG was 17.3% higher in TRT-O compared to CON-O. Although the DMI was statistically significant, the numerical difference was small, and the FCR was 16.4% lower in TRT-O than in CON-O, but the difference was not significant. During the entire test period, the average ADG was higher in the TRT-O group than in the CON-O group (p < 0.02), and the FCR was also improved (p < 0.03).
The effects of increased CP intake during pregnancy on carcass traits of male offspring of Hanwoo cattle are shown in Table 7. Carcass weight increased by 57.3 kg in TRT-O compared to CON-O (p <0.01), and back fat thickness also increased in TRT-O. The rib eye area was similar between the treatments, and the yield index (p < 0.02) and yield grade score (p < 0.02) were higher in CON-O than in TRT-O. The marbling score was 14.2% higher in TRT-O than in CON-O, but there was no significant difference. Meat color, fat color, texture, maturity, quality grade score, and auction cost also showed little difference between the treatments.
The effects of increased CP intake during pregnancy on economic efficacy in Hanwoo cattle are shown in Table 8. Gross receipts were significantly increased in TRT-O compared with CON-O (p < 0.04), and feed costs also showed a significant increase in TRT-O, but the numerical difference was not significant (p < 0.01). Net income increased by an average of 1,331 USD/head in TRT-O compared with CON-O (p < 0.04).
2) Commercial concentrate: pregnant cows, 0.32 USD/kg; top dressing, 0.34 USD/kg; calf, 0.36 USD/kg; growing, 0.34 USD/kg; early fattening, 0.35 USD/kg; late fattening, 0.36 USD/kg; roughage: timothy, 0.53 USD/kg; rice straw, 0.21 USD/kg.
3) Other: water, Power and Fuel cost (32.78 USD), Veterinary and medicine cost (29.30 USD), Automobile cost (40.30 USD), Farm implements cost (167 USD), Farm building and facilities cost (122 USD), Miscellaneous materials cost (73.87 USD), Interest on borrowed capital (60.36 USD), Land rent (4.34 USD), Hired labor cost (44.44 USD), Excretion disposal cost (22.90 USD), Production management cost (18.28 USD).
DISCUSSION
Fetal growth depends on nutrients received during pregnancy. In cattle, fetal organogenesis simultaneously with placental development. A heartbeat can be detected within at least 21 days, and the extremities begin to develop at 25 d of pregnancy, followed by the development of vital organs, including the liver, lungs, brain, and kidneys. In addition, Chalk [6] reported that the development of ovaries and testes begins at 60 d of age. Mao et al. [18] reported that significant kidney growth occurs during mid-pregnancy, and accelerated liver and heart growth occurs during late pregnancy. In ruminants, most fetal structural growth occurs in the last third of the pregnancy period [19], where important organs and tissues are established within a few months, while 75% of fetal growth occurs 2 months before delivery [20]. Mao et al. [18] found that the weight of the semitendinosus muscle of the fetus increased from 214- to 483-times at 9 months compared to that at 3 months of pregnancy, depending on the breed. The fat-weight to body-weight ratio and the total body fat increased continuously throughout the pregnancy period. Ferrell et al. [21] also reported that cattle fetuses undergo rapid growth during the middle to late stages of pregnancy. Similar to the findings of previous studies, our results showed that Hanwoo cattle fetuses grew rapidly between 180 and 270 d of pregnancy and remarkably during the late stages of pregnancy. Because the vital organs for maintaining the life of the fetus during early pregnancy are relatively unaffected by maternal nutritional status [22,23], overall growth is presumed to be dominant in the late stage of pregnancy because vital organs are a higher priority for nutrient distribution than skeletal muscle and fat in early pregnancy [24]. Because the growth rate of the fetus increases after the middle stages of pregnancy, the mother requires additional nutritional supplementation to supply sufficient nutrients to the fetus.
The highest developmental periods of organogenesis, myogenesis, and adipogenesis in the fetus differ throughout gestation [9,18,25]. Fetal muscle development involves 2 processes: an increase in muscle cell numbers (hyperplasia) and their size or diameter (area and length), known as hypertrophy [26]. Primary myogenesis occurs during early pregnancy with minimal skeletal muscle development. Secondary myogenesis occurs during mid-pregnancy, and skeletal muscles develop in earnest [6]. The total number of muscle cells in the fetus is fixed by 240 days of pregnancy, and the area and length of the muscle increase in the second half of the pregnancy period [27]. The number, type, and size of muscle fibers are the main factors that determine muscle mass, and the number of muscle fibers is positively correlated with the potential for growth after delivery [28].
Myogenesis is a complex process involving the proliferation and differentiation of precursor cells and is affected by various genes and myogenic regulatory factors, including MyoG [24]. MyoG is an important transcription factor involved in stimulating muscle growth by inducing myogenesis and contributing to the formation of muscle cells [29]. In addition, MyoG is directly involved in muscle growth (muscle hypertrophy) and affects carcass weight [30,31]. Myosin heavy chain is involved in muscle contraction and force generation [32]. Four isoforms have been reported in mammalian skeletal muscles [33]. MYH 1, which has low ATPase activity and slow contraction action, is necessary for posture maintenance and is mainly found in the red muscles [33]. MYH 2A is abundant in constantly used muscles and is highly metabolic due to oxidation and glycolysis processes.
In this study, the fetal muscle cell size increased throughout the pregnancy period, and the expression of genes (MYH 1, MYH 2A) related to muscle contraction (motility) was higher than that of muscle cell differentiation (MyoG). Similar to our findings, Duarte et al. [1] found no difference in the mRNA expression of MyoG in the skeletal muscle of the fetus during the gestation period (150 to 240 d). Picard and Gagaoua [26] reported that metabolic and contractile maturation of muscle fibers mainly occurs in the last trimester of pregnancy. Therefore, muscle generation is dominant from the beginning to the middle stages of pregnancy, and metabolic processes related to muscle hypertrophy and contraction are actively generated after the middle stage of pregnancy.
Adipose tissue is vital for energy storage, systemic metabolism, homeostasis regulation, and endocrine and paracrine signaling. Adipose tissue is formed during the prenatal period and develops during the late stages of pregnancy. Du et al. [9] reported that fetal adipocytes were produced before and after 4 months of gestation and lasted up to 8 months after delivery. Chalk [6] also reported that myogenesis and adipogenesis are derived from the same mesenchymal stem cells and that more mesenchymal stem cells are used for adipogenesis in the later stages of pregnancy. In a study by Mao et al. [18], fetal body fat increased continuously from 3 to 9 months of pregnancy, resulting in a significant increase in the total fat to body weight ratio.
Adipocytes grow by receiving nutrients and oxygen from blood vessels and are surrounded by an extensive capillary network [34,35]. As the formation of new blood vessels is required to grow and develop adipose tissue, angiogenesis occurs in adipose tissue [36,37]. Capillary endothelial cells interact with fat cells through paracrine signaling pathways, extracellular components, and direct cell-cell interactions [34,38,39]. In addition, activated adipocytes produce angiogenic factors such as vascular epidermal growth factor, fibroblast growth factor 2, leptin, and hepatocyte growth factor, which, alone or in cooperation, increase angiogenesis and promote the growth and metabolism of adipose tissue [40]. Consequently, the growth and development of adipose tissue are highly dependent on angiogenesis. In this study, the formation of adipocytes around blood vessels in fetal skeletal muscle was observed at 180 d of gestation. Therefore, intramuscular adipocytes in the fetus can be formed in the middle stage of pregnancy, similar to other adipose tissues. It is assumed that their formation around the blood vessels increases interaction with blood vessels to obtain a supply of nutrients.
Differentiation of mature adipocytes is regulated by transcription factors such as the C/EBP family and PPARγ [41]. C/EBPβ not only regulates the expression and activity of PPARγ but is also involved in the synthesis of PPARγ ligands [42]. Adipocyte differentiation is also caused by stem cells present in the muscle [43], and the proliferation, differentiation, and size of adipocytes in the muscle are closely related to the marbling score [43,44]. Mitochondria use energy and fatty acids to form acetyl-CoA, which is used as a precursor for intramuscular fat synthesis [45], and acetyl-CoA synthesizes long-chain fatty acids (saturated fats) through the action of various enzymes. SCD converts saturated fatty acids into monounsaturated fatty acids, allowing fat accumulation [46]. In this study, the expression of SCD and PPARγ was high in LD muscle at 180 d of gestation, and the expression of C/EBPβ continuously increased throughout the pregnancy period. Similarly, in a study by Duarte et al. [1], the mRNA expression of PPARγ in fetal skeletal muscle was higher at 190 d of gestation than at 135 and 240 d of gestation. In addition, the timing at which the expression of these genes increased and the timing of intramuscular adipocyte discovery coincided. Therefore, SCD, PPARγ, and C/EBPβ are important genes related to the differentiation of intramuscular fat during the prenatal stage. Since the expression of C/EBPβ in fetal skeletal muscle and the area of intramuscular adipocytes continuously increased, it was determined that the differentiation of intramuscular adipocytes was maintained from the middle stages of pregnancy until delivery.
Nutrients consumed by pregnant cows are vital for maternal maintenance and growth, fetal development, and postpartum recovery. In this study, increased CP intake did not affect pre- or postpartum cow weight or subsequent fertility (Table 5). Wilson et al. [47] reported that there was no difference between pre- and postpartum (115 d) BW, AI conception, and overall pregnancy in a 129% treatment group compared to the 100% protein requirement group. Some studies did not show an effect on pre- and postpartum BW and subsequent reproduction according to protein supplementation for a period of time [48, 49, 50]. However, in a study by Valiente et al. [51], cow BW and ADG were higher in heifers with 121% of protein requirements (HP) than in 64% of protein requirements (LP) without any difference in subsequent reproduction. Funston et al. [52] and Martin et al. [53] reported similar results. Unlike previous studies using cows, heifers continue to grow physiologically, so it is judged that protein supplementation during pregnancy can affect body growth.
Excessive increased CP intake in cows increased the blood urea nitrogen concentration, negatively affected the uterine environment, and reduced reproductive function [54]. Since there was no difference in SPC and conception rates between treatments in this study, it is speculated that increase 5% CP intake does not adversely affect subsequent reproduction. Similar to our study, Stalker et al. [50] found that protein supplementation (0.45 kg/cow per d, protein 42%) during pregnancy did not affect cows’ subsequent reproductive performance and fertility rates.
Controlling maternal dietary protein intake during pregnancy is essential for embryo survival, growth, and development [11]. It is important to establish adequate nutritional levels because it can have lifelong effects on offspring. In particular, when maternal protein intake is too low or too high, birth BW is reduced because of placental dysfunction and intrauterine growth retardation, which affects postnatal growth and feed efficiency [7,55]. In this study, increased CP intake during pregnancy improved the birth BW, ADG, final BW, and FCR of male offspring (Table 6), resulting in a significant increase in carcass weight (Table 7). Beaty et al. [56] reported that the weaning weight of calves improved when the supply of protein increased during pregnancy, and Underwood et al. [5] support our findings by reporting that male offspring born on improved pastures (protein 6% to 11%) have increased weaning BW, final BW and carcass weight compared to offspring born on native pasturelands (protein 5.4% to 6.5%). Carvalho et al. [57] reported that protein supplementation (CP 40%, 3.5 g/kg of body weight) from mid-pregnancy a positively affected the energy metabolism and favored the hypertrophic processes of the skeletal muscle in offspring. In addition, there are reports that birth BW and offspring growth are improved by supplementation with protein before delivery [1,51]. On the other hand, there is evidence that protein restriction during pregnancy negatively affects muscle development of offspring [58-60]. In other words, the level of protein intake during pregnancy is considered an important factor in growth of offspring. The various amino acids in the protein stimulate the secretion of growth hormone and insulin and regulate protein synthesis in skeletal muscle and placenta by enhancing oxidative and cellular redox capacity [61]. It has also been shown to promote blood vessel angiogenesis and growth, improve blood flow across the placenta, and increase maternal-fetal nutrient transfer [7, 62]. It has been speculated that these effects may eventually positively affect fetal development and postnatal growth. In addition, birth weight is highly related to weaning weight and ADG [63]. Song et al. [64] analyzed the data of 1,907 steers and reported that birth weight was significantly positively correlated with weaning weight, carcass weight, and rib eye area. Therefore, the increase in birth weight due to increased CP intake during pregnancy may have affected final weight and carcass weight. However, in a study by Wilson et al. [47], protein supplementation (128% of protein requirement) for 78 d before delivery did not affect the birth BW and growth of the offspring. Therefore, it was judged that the performance of the fetus might differ depending on the time of protein supplementation, which may be related to the developmental sequence of muscle fibers and adipose tissue according to the growth stage of the fetus [9].
It is well known that stromal vascular cells are a major source of adipogenic cells in skeletal muscle [65,66], and proteins influence fetal angiogenesis [11,67]. We hypothesized that increased CP intake during pregnancy could improve proliferation of intramuscular adipocytes in fetal. However, there was no difference in the degree of intramuscular fat in male offspring with or without increased CP intake in this study, but rather a significant difference in back fat thickness. Similar to our study, offspring supplemented with better protein during pregnancy showed increased back fat thickness and number of subcutaneous fat cells (cells/field) without any difference in intramuscular fat and increased insulin sensitivity [5,47]. However, in a study by Larson et al. [10], supplementation of protein cubes (0.45 kg/d dry matter, protein 28%) during winter grazing of pregnant cows improved the intramuscular fat content of the offspring. Recent evidence suggests that intramuscular adipocytes behave differently from subcutaneous and visceral adipocytes [68-71]. Moreover, intramuscular adipocytes share a common progenitor cell with myogenic cells and are in a competitive relationship [72]. It is presumed that extreme nutritional deficiencies, such as winter grazing, induce the differentiation of fetal intramuscular adipocytes, which may have a greater fetal programming effect, but this is inaccurate. There is evidence that increased CP intake during pregnancy can improve adipogenesis in offspring. However, the mechanisms regulating the differentiation of intramuscular adipocytes have not yet been established, and this should be further discussed and studied.
Fetal programming through maternal nutrition can improve offspring performance and provide economic benefits. In this study, although the costs of commercial concentrates for pregnant cows and male offspring increased slightly, increased CP intake during pregnancy increased gross receipts and net income by improving the carcass weight of the offspring. Larson et al. [10] reported that the USDA choice ratio and carcass weight of the offspring increased due to protein supplementation during the pregnancy period, resulting in an increase of $30/head revenue; Summers et al. [73] reported that the feedlot purchase price increased because the calf’s weight increased with dried distiller’s grain with solubles-based protein supplementation. In addition, Stalker et al. [50] reported that maternal protein supplementation increased weaning BW and survival rate of calves, resulting in increased net income. Studies have shown that increased CP intake of maternal can improve the growth and BW of offspring, thereby increasing their economic benefits.
CONCLUSION
Through our study, it is judged that adipogenesis begins in fetal skeletal muscle in at least mid-pregnancy (4 to 6 months). Based on this, a scientific approach is needed to produce high-quality beef through fetal programming effectively. While excessive nutrient over nutrition during pregnancy can negatively affect offspring, 5% increased CP intake during pregnancy can increase profitability through higher meat production yield (carcass weight) by improving the growth of the fetus and offspring. Simultaneously, there is a possibility of increasing subcutaneous fat rather than intramuscular fat; therefore, additional research should be conducted to compensate for these problems.