INTRODUCTION
The intestinal epithelium digests and absorbs nutrients and forms a barrier against pathogens and toxic substances [1,2]. The intestinal epithelium is also important for maintaining health as it is the first physical barrier to detect external substances [3]. Damage to the intestinal epithelium disrupts immune homeostasis, increases inflammation, disrupts its function as a barrier and leads to many intestinal diseases, including pathogenic infections [4,5]. The intestinal epithelium is constantly exposed to bacteria, fungi, viruses, and parasites that can act as pathogens [6]. The intestinal epithelium is exposed to higher concentrations of mycotoxins than other organs, thus, it is the first to suffer toxic damage [7,8]. Exposure of the intestinal epithelium to mycotoxins disrupts its function as a barrier through disrupted intestinal integrity (physical), thinned mucus layer (chemical), imbalanced inflammatory factors (immunological), and dysfunctional bacterial homeostasis (microbial) [8].
Deoxynivalenol (DON) is a mycotoxin produced by Fusarium species and contaminates food and feed worldwide [9]. DON mainly contaminates crops such as maize, wheat, and barley and exposure to it poses a health threat to both humans and animals [10]. Pigs are the most susceptible animals to DON [11]. After eating DON-contaminated feed, they suffer from anorexia, vomiting, diarrhea, and deterioration of their immune and reproductive functions [12]. Additionally, DON affects growth and weight gain rate, and causes stiff pig syndrome, abortion, stillbirth, and weak offspring [13]. Current methods to reduce exposure to mycotoxins include thermal processing, chemical agents, and toxin binders [14]. However, these methods do not completely destroy mycotoxins, and toxin binders change their nutritional value and reduce bioavailability of trace minerals or vitamins [15]. Therefore, research on other natural products that can alleviate mycotoxins is required.
Lauric aci (LA) is a medium-chain fatty acid (MCFA) composed of 12 carbon atoms and is found in coconut oil, milk, and black soldier flies (BSF, Hermetia illucens L.) [16,17]. MCFAs, including LA, influence nutritional functions, digestion, absorption, and energy supply in monogastric intestines, while also regulating immune responses, relieving stimulus-induced symptoms, and contributing to barrier functions [18–20]. Therefore, LA, one of MCFAs, can be used as an inexpensive and new feed additive to replace antibiotics [20,21]. Nevertheless, the molecular mechanisms by which LA benefit the mycotoxin-damaged small intestine epithelium are unknown and require further study. Therefore, in this study, we investigated the molecular mechanism of LA in mitigating DON-induced dysfunction in porcine small intestinal epithelium using IPEC-J2 cells.
MATERIALS AND METHODS
IPEC-J2 cells (DSMZ, Braunschweig, Germany) isolated from unsuckling piglet jejunal epithelium were cultured in Dulbecco’s Modified Eagle Medium (Thermo Fisher Scientific, Wilmington, DE, USA) supplemented with 10% fetal bovine serum and 1% penicillin-streptomycin in a 37°C CO2 incubator. Lauric acid (Sigma-Aldrich, St. Louis, MO, USA) was diluted with dimethyl sulfoxide for treating IPEC-J2 cells.
IPEC-J2 cells were seeded in 3 × 104 cells in a 96-well plate, cultured for 24 h, before they were exposed to LA (0, 0.1, 0.2, 0.3, 0.4, and 0.5 mM) or LA (0.2 mM) and DON (0.25 μg) for 24 h. After 2 h of treatment with Water-Soluble Tetrazolium 1 (WST-1) (Roche Diagnostics GmbH, Mannheim, Germany), cell viability was measured by analyzing the absorbance of the dye by subtracting the background wavelength from 450 nm to 600 nm using a GloMax Discover Multi-Microplate Reader. And Cell viability was compared to control group and was calculated by converting absorbance to percentage.
After 24 h of LA and DON treatment, IPEC-J2 cells were harvested, washed with phosphate-buffered saline (PBS), and centrifuged. After discarding the supernatant, 1X Annexin binding buffer 5 μL Alexa Fluor 488 Annexin-V (Thermo Fisher Scientific), and 1 μL 100 mg/mL propidium iodide (PI) working solution were added. After incubation of the cells at room temperature for 15 min in the dark, the nucleus was stained with 4’,6-diamidino-2-phenylindole (DAPI;Vector Laboratories, Burlingame, CA, USA) and mounted on a slide using a coverslip. Pictures of the cells were taken using a fluorescence microscope (Korealabtech, Seongnam, Korea).
IPEC-J2 cells treated with LA and DON were grown on gelatin-coated glass cover slips for 24 h before they were fixed with 4% paraformaldehyde for 15 min. After that, the cells were treated with a blocking buffer for 1 h. Rabbit anti-forkhead box O3 (FOXO3a) IgG was treated with a 1:200 antibody solution and probed overnight. After washing with PBS three times for 3 min, goat antirabbit (488) (Thermo Fisher Scientific, Carlsbad, CA, USA) was treated with the antibody solution at a ratio of 1:500 and incubated for 1 h in the dark. Thereafter, the nucleus was stained with DAPI (Vector Laboratories) covered with a cover slip, the cells were photographed under a fluorescence microscope.
Cytoplasmic and nuclear proteins were extracted using NE-PER Nuclear and Cytoplasmic Extraction Reagent (Thermo Fisher Scientific) according to the manufacturer’s instructions. Cells were washed with chilled PBS and centrifuged at 500×g for 3 min. After discarding the supernatant, cytoplasmic extraction reagent I (CERI) was added to the cell pellet, vortexed for 15 s before the suspension was incubated on ice for 10 min. Cytoplasmic extraction reagent II (CERII) was added and vortexing for 15 s, the mixture was incubated on ice for 1 min. After centrifugation of the suspension at 16,000×g, the cytoplasmic extraction suspension was transferred to a prechilled tube. A nuclear extraction reagent (NER) was added to the insoluble pellet before vortexing for 15 s every 10 min for a total of 40 min. After centrifugation at 16,000×g for 10 min, the supernatant was transferred into a prechilled tube. The extracted protein was analyzed for protein concentration using the Pierce Bicinchoninic acid (BCA) Protein Assay Kit (Thermo Fisher Scientific). The extracted proteins were used in subsequent analyses.
Protein samples were denatured in 4X Laemmli buffer (4% sodium dodecyl sulfate, 10% 2-mercaptoethanol, 20% glycerol, 0.004% bromophenol blue, and 0.125 M Tris-HCl) (3:1) at 95°C for 5 min. Proteins were electrophoretically separated on 12% sodium dodecyl sulfate-polyacrylamide gel electrophoresis (SDS-PAGE) gels (run for 1 h at 100 V) before they were transferred to polyvinylidene fluoride membranes (Thermo Fisher Scientific). After blocking for 2 h, the proteins were treated with anti-FOXO3a (Novus Biologicals, Centennial, CO, USA) antibody overnight. The membrane was washed three times with Tris-buffered saline containing Tween 20 (TBST, 20 mM Tris, pH 7.5, 150 mM NaCl and 0.1% Tween 20) for 10 min before it was treated with a secondary antibody for 1 h at room temperature. Protein bands were visualized for immunoreactivity using the Enhanced Chemiluminescence (ECL) reagent, and protein bands were imaged using the ChemiDoc imaging system.
Total RNA extraction was performed using the AccuPreP Universal RNA Extraction kit (BioNEER, Daejeon, Korea). cDNA was synthesized from total RNA (1 μg) using the DiaStar™ RT Kit (SolGent, Daejeon, Korea). Primer 3 (http://frodo.wi.mit.edu) was used to design primers for target genes in qPCR. For qRT-PCR, 40 cycles of 95°C for 3 min, 95°C for 15 sec, 56°C–58°C for 15 sec, and 72°C for 15 s were used. Levels of target genes were calculated using the 2−ΔΔCt method, normalized to glyceraldehyde-3-phosphate dehydrogenase (GAPDH). The primer sequences of the genes are shown in Table 1.
The general linear model (PROC-GLM) procedure in SAS was used to test for significant differences between treatment groups. Fig.1B Data were analyzed using t-tests. Symbols of significance were set as **p < 0.01, ***p < 0.001. For other cell viability and PCR data were evaluated for significant differences between treatment groups using Duncan’s multiple range test. All experiments were performed independently in triplicate.
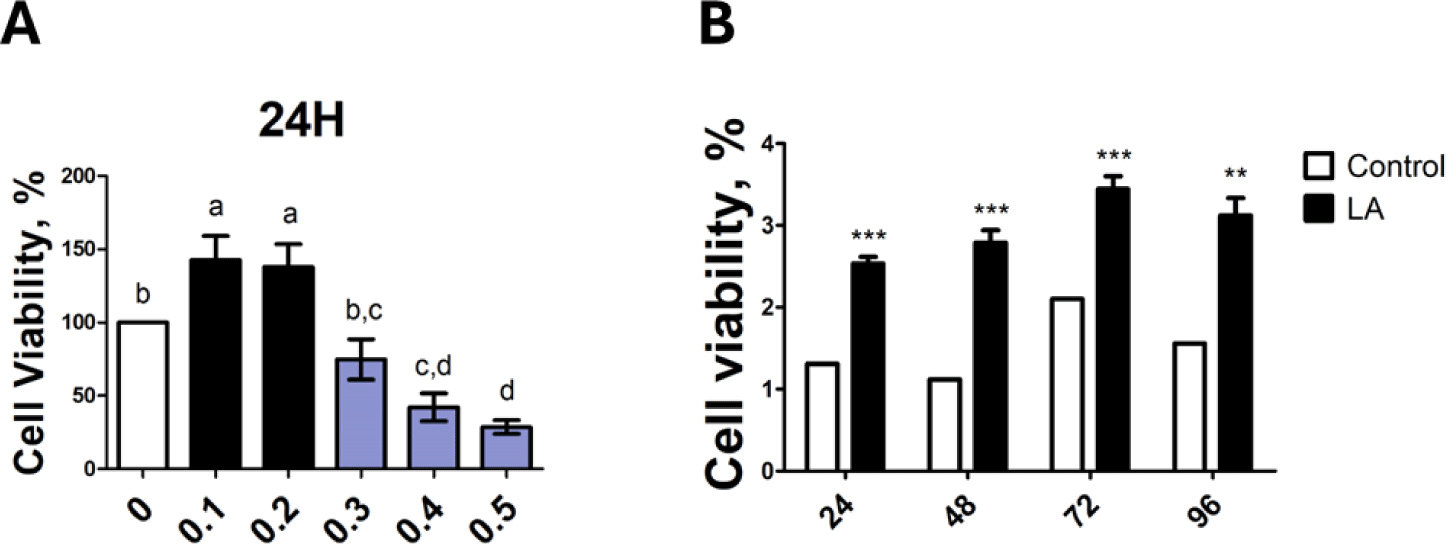
RESULTS
To confirm the cell viability of LA for IPEC-J2 cells, the cell proliferation analysis WST-1 assay was performed. IPEC-J2 cells were exposed to LA at different concentrations (0, 0.1, 0.2, 0.3, 0.4, and 0.5 mM); Concentrations of 0.1–0.2 mM of LA increased cell proliferation whereas LA at concentrations of 0.3 mM or higher decreased cell proliferation (Fig. 1A). Therefore, LA concentration of 0.2 mM was chosen for further analyses. The effect of 0.2 mM LA on IPEC-J2 cells was time dependents cell proliferation increased at 24, 48, 72, and 96 h (Fig. 1B). Accordingly, we used 24 h for further analyses. Cell viability was compared between all treated and control groups by converting absorbance values into percentages as shown in the figure.
We used the cell proliferation analysis WST-1 assay to assess whether LA affects cell proliferation capacity induced by DON. IPEC-J2 cells treated with DON showed significantly lower cell proliferation than those in the control. However, we observed that when DON-treated IPEC-J2 cells were co-treated with LA, cell proliferation increased (Fig. 2), indicating that LA alleviates DON-induced reduction in cell proliferation capacity.
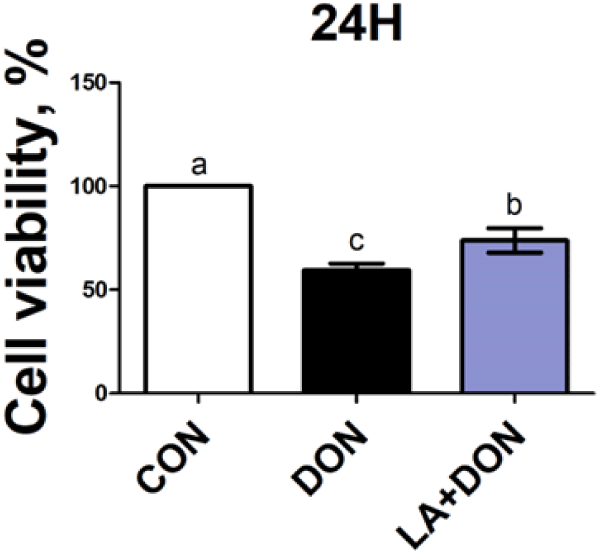
To determine whether LA alleviates DON-induced apoptosis and necrosis in IPEC-J2 cells, we performed double staining with Annexin-V and propidium iodide. Apoptosis and necrosis were significantly increased in IPEC-J2 cells treated with DON. In contrast, when IPEC-J2 cells exposed to DON were treated with LA, apoptosis and necrosis were reduced (Fig. 3A). Additionally, when examining the mRNA expression levels of apoptosis-related genes such as Bcl-2, Bcl-6, CASP3, and CASP9, the mRNA expression was significantly lower when cells were exposed to both LA and DON than to DON alone. Moreover, the mRNA expression of FOXO3a was also higher in cells exposed to DON than in those in the control and decreased when cells were treated with LA (Fig. 3B)., indicating that LA alleviates DON-induced apoptosis and necrosis.
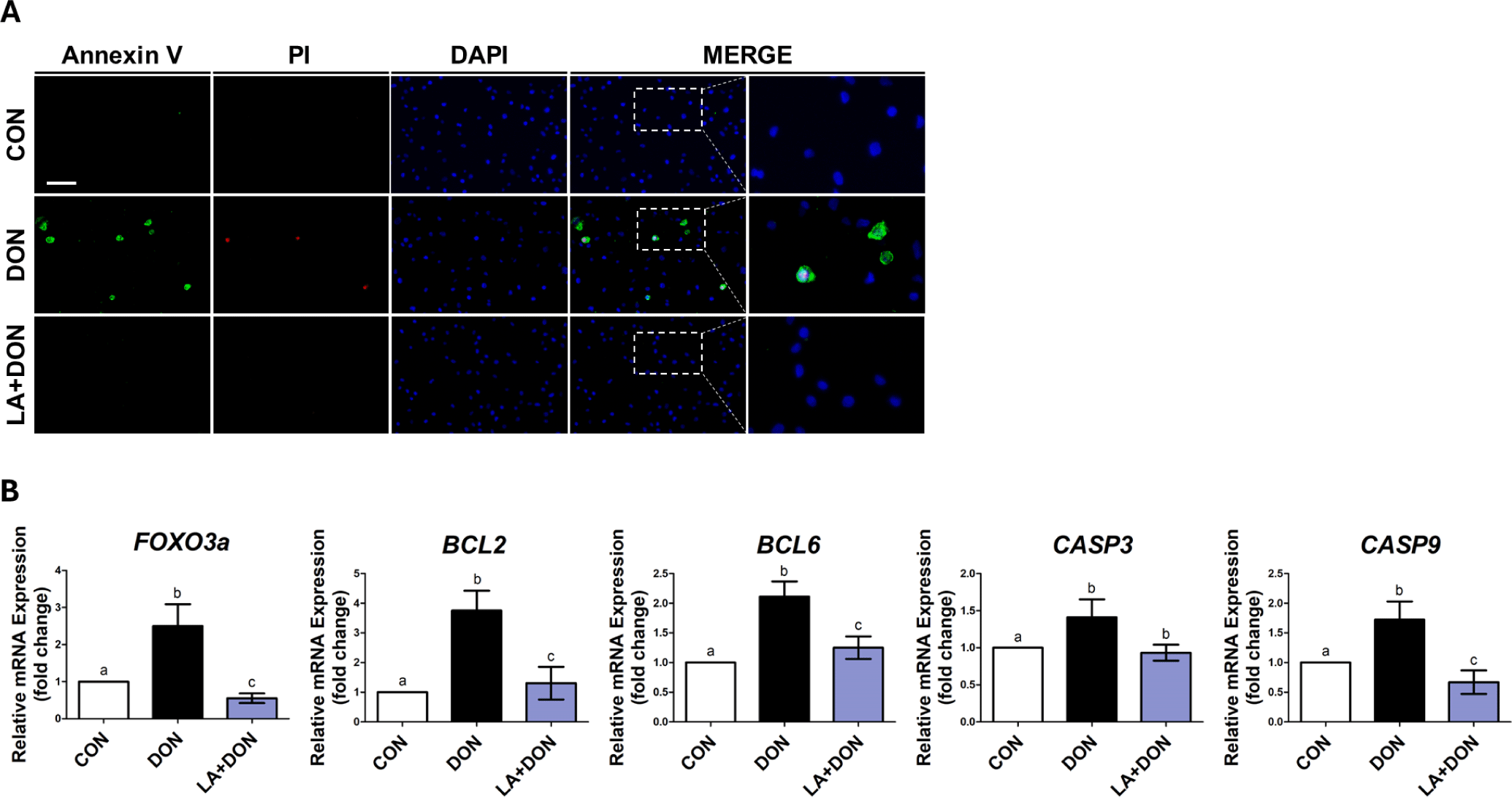
Annexin-V results showed that LA alleviated DON-induced apoptosis and necrosis. We hypothesized that this might be influenced by FOXO3a signaling. Therefore, we measured the expression of FOXO3a protein in IPEC-J2 cells using immunocytochemistry, Western blotting, and real-time PCR. Immunocytochemistry results showed that DON-induced FOXO3a nuclear translocation was similar to that of the control. However, DON-induced FOXO3a nuclear translocation was attenuated in cells co-treated with DON and LA (Fig. 4A). Additionally, Western blotting results showed that the expression of FOXO3a was significantly decreased in the cytoplasm and significantly increased in the nucleus of DON-treated IPEC-J2 cells. In contrast, in IPEC-J2 cells co-treated with LA and DON, the expression of FOXO3a significantly increased in the cytoplasm and significantly decreased in the nucleus (Fig. 4B).
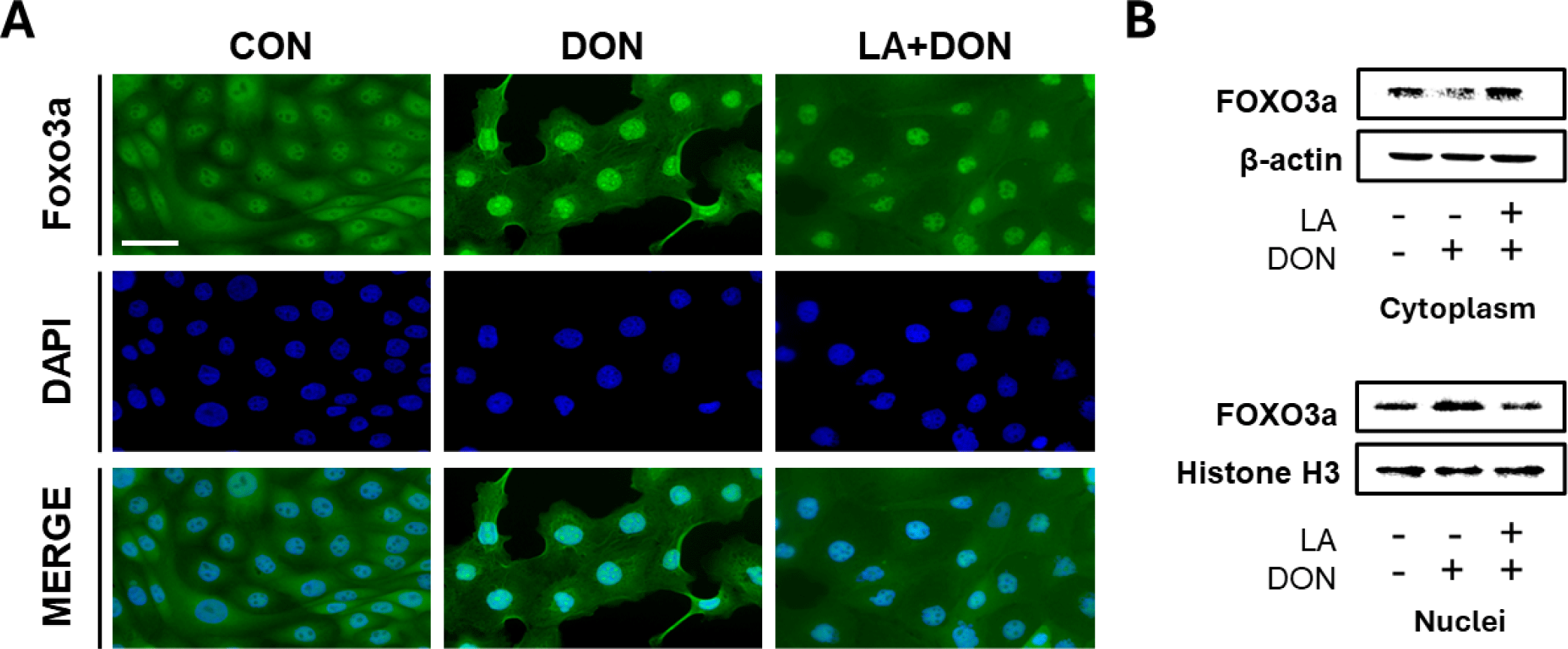
DISCUSSION
DON is a mycotoxin that causes various symptoms such as anorexia, vomiting, and stunted growth, leading to poor health and economic loss [22]. The intestine is the first biological barrier to mycotoxins because it is the first target of exposure after consuming contaminated food [23,24]. Intestinal exposure to DON causes symptoms such as destruction of intestinal epithelial cells, impairment of integrity of intestinal barrier, destruction of tight junction protein structures, increased intestinal epithelial permeability, reduced nutrient absorption and transport efficiency, inflammation, and induction of apoptosis [25]. To reduce exposure to mycotoxins, various methods such as toxin binders are used, but they are not completely effective [14,15]. LA is a MCFA that promotes intestinal health through its effects such as antibacterial activity, reduction of inflammatory response, and reduction of oxidative stress [18,26]. Previous studies have shown that LA has beneficial effects on the intestine through its antibacterial activity [27–29]. Therefore, LA can be used as a new feed additive to replace antibiotics. Thus, we speculated that LA would alleviate DON-induced damage to the small intestine epithelial cells.
First, we investigated the cytotoxicity of various concentrations of LA to IPEC-J2 cells. The WST-1 assay showed that LA concentrations of 0.1 and 0.2 mM had a beneficial effect on cell proliferation. However, there was no statistically significant difference between 0.1 and 0.2 mM in cell viability. Therefore, further analyses were conducted using an LA concentration of 0.2 mM. In a previous study, when IPEC-J2 cells were exposed to DON at a concentration of 250 ng/mL, cell proliferation was significantly lower than that of the control group [30]. Therefore, we decided to use DON at a concentration of 250 ng/mL for further analyses. We observed that cell proliferation was significantly higher in cells co-exposed with LA at 0.2 mM and DON than in cells exposed only to DON 0.25 ug/mL.
Apoptosis is a highly regulated cell death process induced by various stimuli such as mycotoxins and unfavorable physiological conditions, which can damage the intestinal epithelium and lead to intestinal pathology [31–33]. Reduced cell proliferation is associated with increased apoptosis [34,35]. Therefore, we speculated that cell proliferation reduced by DON and increased when treated with LA might also be related to apoptosis. FOXO3a induces apoptosis by inhibiting the apoptosis-inhibitor protein [36]. It also induces the expression of several proapoptotic members, including Bcl-2 and Bcl-6, to inhibit cell growth and promote apoptotic signaling [33,37]. Expression of these proapoptotic proteins catalyzes the activation of the initiator caspase (caspase-9), which in turn activates the effector factor (caspase-3) [38,39]. Activated caspase-3 causes morphological changes in apoptotic cells [39]. For example, caspase-3 cleavage of caspase-activated DNAse (ICAD) initiates activation of caspase-activated DNAse (CAD). The activation of CAD then cleaves the DNA at the inter-nucleosome linker sites, leading to chromatin condensation and DNA fragmentation [40,41]. This form of DNA fragmentation is a common characteristic of cells undergoing apoptosis. In addition, Bcl-2 is overexpressed to prevent cell death in the early stages of necrotic activation [42]. Our RT-PCR results showed that the mRNA expression of FOXO3a was increased in cells exposed to DON but decreased in those exposed LA. Also, genes related to apoptosis such as Bcl-2, Bcl-6, CASP3, and CASP9 were increased by DON and decreased by LA relative to the control group. Additionally, we observed that LA alleviated DON-induced apoptosis and necrosis through Annexin-V staining. Therefore, our findings confirmed that LA mitigated DON-induced apoptosis and necrosis in IPEC-J2 cells.
The forkhead box O (FOXO) protein activates or inhibits the transcription of target genes through its DNA-binding foxo domain in the nucleus via the nucleocytoplasmic shuttle, and regulates cell cycle arrest, DNA repair and apoptosis, stress, and tumor suppressor pathways [43]. FOXO family members of the Forkhead transcription factor include Foxo1, Foxo3, Foxo4, and Foxo6 [44]. Among several FOXO family members, FOXO3a is abundant in various tissues including the brain, heart, kidney, and spleen and is the most important transcription factor involved in apoptosis and cell cycle inhibition [33,43]. Upon intestinal exposure to factors such as mycotoxin, FOXO3a protein translocates from the nucleocytoplasm to the nucleus to form DNA-binding foxo domains, triggering apoptosis and leading to intestinal damage (Fig. 5A). Our immunocytochemistry and Western blotting analysis showed that DON treatment induced nuclear translocation of FOXO3a protein. In contrast, DON-induced nuclear translocation of FOXO3a protein was attenuated when cells were co-treated with DON and LA (Fig. 5B). Our results show that LA reversed the increase in apoptosis caused by DON-induced nuclear translocation of the FOXO3a protein in IPEC-J2 cells.
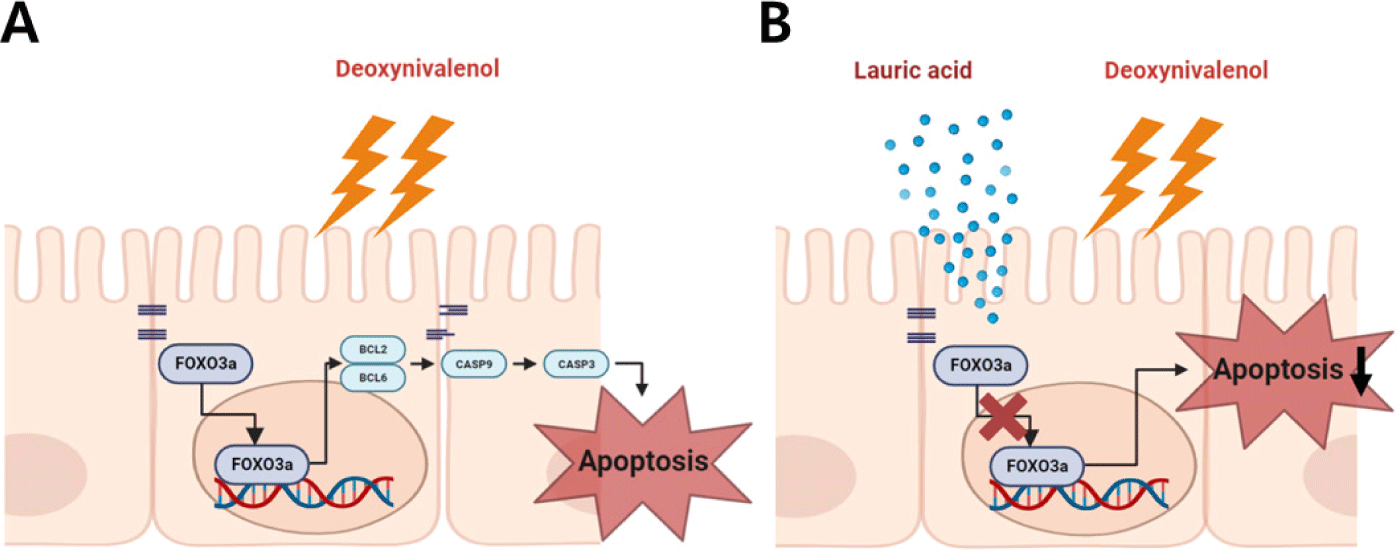
This study is the first to investigate the effect of LA on DON-treated porcine intestinal epithelial cells. Our findings indicated that LA alleviated DON-induced reduction in cell proliferation and DON-induced increase in apoptosis (Figs. 5A and 5B). This suggests that LA has a beneficial effect on DON-induced damage to the intestinal epithelium. Additionally, our results suggest that LA can be used as a natural antibiotic in the future.