INTRODUCTION
Pig production is an economically important global industry [1]. A recent report from the European Union (EU) revealed that, in 2018, the pig production industry produced 148 million pigs, making pigs the largest livestock category in the region [2]. To meet the increasing demand, strategies for pig production have been developed to improve production yield, meat quality, profitability, and sustainability [3]. However, producers still face challenges related to increasing feed costs, poor growth performance, and the occurrence of diseases in pigs. Meanwhile, researchers have found a delicate relationship between the gut microbiome and the health and nutrition of pigs [4–6]. Consequently, the gut microbiome is a viable target for improving livestock production and increasing economic efficiency [3,4]. Dietary intervention is currently one of the primary strategies for targeting the gut microbiome of pigs. Recent evidence suggests that dietary intervention can modulate the gut microbiome and its metabolites and improve health and performance in pigs [7–9]. Optimization of feed and feed supplements has also resulted in increased survival rates of weaning piglets [10]. During the past decades, our knowledge of the role of gut microbiome in swine health and nutrition has expanded, from simple enhancement of nutrient absorption to the regulation of neurological functions. One of the mechanisms by which the gut microbiome affects the overall health of the host is through the production of metabolites [9,11]. Host recognition of microbiome-derived metabolites is integral to regulating and promoting pig health [6,11].
After the EU ban on antibiotic use in livestock production, dietary supplementation with probiotics has become a promising alternative to improve pig health and productivity [12,13]. Probiotic feeding has been shown to modulate the gut microbiome and alter its metabolite levels [9,14]. Moreover, it can also benefit livestock production, although some results have been inconsistent [12,15,16]. To date, our knowledge of gut microbiome-derived metabolites and their functional activities is limited, and the role of probiotics in modulating these metabolites is not well understood. In this review, the role of gut microbiome-derived metabolites in swine health and performance are discussed. In addition, this review summarizes recent studies demonstrating the effect of probiotics on these metabolites and their potential impact on pig production.
GUT MICROBIOME AND ITS METABOLITES
In recent years, our knowledge regarding the gut microbiome has greatly expanded owing to advancements in DNA sequencing technology and the availability of more powerful bioinformatics tools [9,14]. The gastrointestinal tract (GIT) consists of trillions of bacteria belonging to more than one thousand taxonomic groups, most of which are yet to be cultured and identified [11,17,18]. In addition to bacteria; viruses, archaea, fungi, and other eukaryotic microorganisms also reside in the gut of mammalian hosts and perform different functions in the GIT, thus creating an extremely complex and diverse ecosystem [9,11]. Until recently, the gut microbiome was regarded as an additional organ of the body owing to its role in the normal functioning of the host [19]. However, further examination reveals that the gut microbiome can be better identified as a part of a ‘holobiont’ with the host [20,21], and in the past few years, this intricate relationship between the gut microbiome and its host has been described extensively [20]. A bidirectional communication exists between the gut microbiome and the host, ultimately influencing health and disease pathogenesis (Fig. 1) [22,23]. For instance, the gut microbiome aids in nutrient absorption and regulation of intestinal barrier function [24]. The gut microbiome is also responsible for regulating intestinal immune function by stimulating anti-inflammatory regulatory T cells [11,23]. Moreover, the gut microbiome plays a key role in the enterohepatic circulation of bile acids and promotes cholesterol metabolism. The gut microbiome regulates the gut–brain axis, affecting host cognitive function and behavior [17,25,26]. Its modulating effect on host health can be attributed either through direct interaction with mucosal epithelial cells (IEC) or indirectly through the production of metabolites. Short-chain fatty acids (SCFAs), secondary bile acids, polyamines, indoles, and phenols are metabolites produced by gut microbiome during metabolism. Some of these metabolites are exclusively produced by the gut microbiome (such as SCFAs and secondary bile acids), while others are produced in conjunction with host cells. Different groups of commensal microorganisms contribute different metabolites to the overall metabolic pool of the host, and this is mainly due to differences in the fermentation capacities (i.e., different genes producing different enzymes) of each member of the gut microbiome. Thus, microorganisms in the gut do not act individually, but rather form consortia to produce these metabolites. The number and composition of the microbiome demonstrate spatial and temporal heterogeneity across the GIT, and the same is true for their metabolites [9,10,27]. For instance, most of the metabolites produced by the gut microbiome are generated in the colon, as most commensals reside there [28]. In addition, pigs of different ages have different gut microbiome composition [10]. These metabolites interact with the mucosal surface and trigger a host response [10,11]. Conversely, metabolites produced by the gut microbiome also influence other microorganisms in the gut; metabolites can either promote certain groups of microorganisms or inhibit their growth in the GIT [28]. Diet has a significant influence on gut microbiome and their metabolites. Dietary inclusion of fibers and various oligosaccharides has been found to increase the fermentation capacity of the gut microbiome to produce SCFAs [29–33]. Meanwhile, varying levels of crude protein in the diet influence amino acid fermentation by-products [24,34]. The mechanism by which these metabolites are produced, contributing microorganisms, and their impact on swine health are summarized in Table 1, and discussed in the following sections.
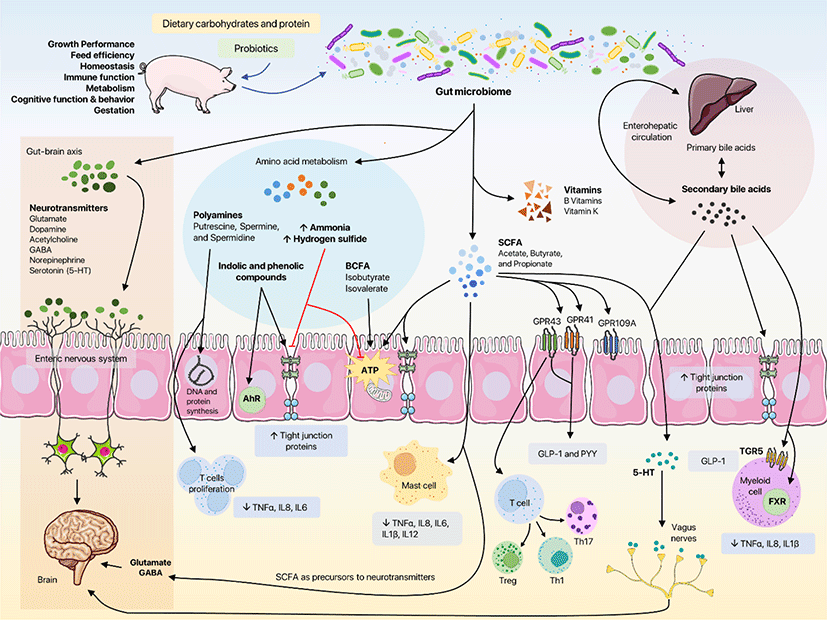
Metabolites | Producing bacteria | Biological functions and effects on pigs | References |
---|---|---|---|
Short-chain fatty acids (acetate, butyrate, and propionate) |
Ruminococcaceae Ruminococcus Lachnospiraceae Blautia Roseburia Lactobacillaceae Clostridium Eubacterium Faecalibacterium Bifidobacterium Bacteroides |
•Energy source for colonocytes •Stimulate cell proliferation •Suppress pro-inflammatory cytokines •Reduce gut permeability through upregulation of tight junction proteins •Regulate lipid metabolism •Act as precursor for neurotransmitters •Improve feed efficiency and lower feed intake •Improve average daily gain •Improve meat quality |
[6,29,33,35–55] |
Branched-chain fatty acids (isobutyrate and isovalerate) |
Clostridium
Propionibacterium Streptococcus Bacteroides |
•Energy source for colonocytes (less preferred) •Suppress pro-inflammatory cytokines (dose-dependent) •Impair gut barrier function •Upregulate pro-inflammatory cytokines |
[56–62] |
Lactate |
Lactic acid bacteria Bifidobacterium |
•Important metabolite for cross-feeding mechanism •Reduce pH thus preventing the growth of potentially pathogenic bacteria |
[6,63,64] |
Bile acids (primary and secondary bile acids) |
Clostridium species Eubacterium Parabacteroides Lachnospiraceae |
•Regulate glucose and energy homeostasis, and lipid metabolism •Suppress pro-inflammatory cytokines •Have antimicrobial and cytotoxic activities •Decrease cell proliferation and gene expression of tight junction proteins •Impair oxidative stress coping mechanism |
[65–74] |
Polyamines (putrescine, spermine,and spermidine) |
Clostridium
Ruminococcus Roseburia Enterococcus Streptococcus Lactococcus Lactobacillus Bifidobacterium Bacteroides Fusobacterium |
•Improve fetal development •Stimulate intestinal maturation •Suppress pro-inflammatory cytokines •Enhance oxidative stress resistance •Increase growth •Alleviate diarrhea •May cause damage to the intestinal morphology |
[75–85] |
Indolic and phenolic compounds |
Clostridium
Peptostreptococcus Lactobacillus Bifidobacterium Escherichia Bacteroides |
•Indole and indole derivatives •Upregulate of aryl hydrocarbon receptors and increased tight junction proteins •Attenuate oxidative stress •Causes ‘boar-taint’ in barrows (skatole) •Phenols and phenol derivatives •Induce ‘leaky-gut’ •Disrupt colonic cell respiration (p-cresol) •Reduce growth performance (p-cresol) |
[60,86–99] |
Ammonia |
Amino acid fermenting commensals Helicobacter |
•By-product of amino acid fermentation •Inhibits mitochondrial respiration •Inhibits short-chain fatty acid oxidation |
[56,87,100,101] |
Hydrogen sulfide |
Clostridium
Fusobacterium Desulfovibrio Enterobacter Escherichia Salmonella |
•Energy source for colonocytes •Acts as a signaling molecule •Inhibits cytochrome oxidase activity •Hinders cell proliferation and induces intestinal inflammation |
[87,88,102,103] |
Neurotransmitters (glutamate, dopamine, acetylcholine, γ-aminobutyric acid, norepinephrine, and serotonin) |
Bacillus
Bifidobacterium Lactobacillus Escherichia Klebsiella |
•Improve cognition and behavior •Ameliorate tail biting |
[104–107] |
Vitamins (B vitamins and vitamin K) |
Bacteroides
Lactobacillus |
•Serve as coenzymes in neurological processes (B vitamins) •Essential vitamin for proper blood clotting (vitamin K) |
[108–110] |
SCFAs are fermentation by-products of indigestible polysaccharides, such as fiber and resistant starch, produced by the gut microbiome [38,111–113]. Amino acid fermentation, on the other hand, yields branched-chain fatty acids (BCFAs) [61,114,115]. Acetate, butyrate, and propionate are the major SCFAs produced in the gut, whereas isobutyrate and isovalerate are the main BCFAs in the GIT [61,114]. Lactate, on the other hand, is produced by lactic acid bacteria and utilized by SCFA-producing microbiota via a metabolite cross-feeding mechanism [63,64]. In pigs and other non-ruminant hosts, SCFA and BCFA concentrations along the intestinal tract exhibit heterogeneity, with the highest concentrations produced in the proximal colon [42,64,115,116]. Absorption of these metabolites occurs mainly in the colon [46]. The mechanism by which SCFAs are produced by the gut microbiome, either by anaerobic fermentation or cross-feeding, has been described extensively in previous publications [38,41,63,117,118]. Dietary fibers and gut microbiome composition greatly modulate these mechanisms [6,119]. Because dietary fibers are complex substrates, their complete degradation requires different members of the gut microbiome working in consortia to produce SCFAs [29,33,113]. The phylum Bacillota (Firmicutes) includes many SCFA-producing members, such as Ruminococcaceae and Lachnospiraceae [37,38]. Faecalibacterium, Eubacterium, Roseburia, Blautia, and Ruminococcus can produce SCFAs, particularly butyrate [29,33,35,36]. Clostridium also participates in the production of SCFAs, particularly acetate, in the swine gut [39]. Members of the phylum Actinobacteria, such as Bifidobacterium, and Bacteroidetes, such as Bacteroides fragilis, contribute to the SCFA pool by producing acetate, propionate, and butyrate [40–42]. Although most major SCFA producers in the gut have been characterized, there could be more unknown gut microbial residents involved in SCFA production. On the other hand, lactate is mainly produced by lactic acid bacteria and bifidobacteria from carbohydrate fermentation [120–122]. Meanwhile, in diets low in fiber, the gut microbiome shifts from SCFA to BCFA production, an adaptive mechanism to yield energy [114]. Clostridium, Propionibacterium, Streptococcus, and Bacteroides are the most common producers of BCFAs [61,62].
In recent years, many studies have demonstrated the role of SCFAs, especially butyrate, propionate, and acetate, in maintaining swine gut health [46]. Butyrate, the major energy source for colonocytes, has been extensively studied because of its anti-inflammatory ability and its effects on energy metabolism and homeostasis [44,45,123]. Butyrate also enhances enterocyte proliferation and reduces apoptosis [46]. Zhong et al. reported that enrichment of Lactobacillaceae and Ruminococcaceae also elevates butyrate concentrations, which in turn regulates gut homeostasis by stimulating cell proliferation and suppressing pro-inflammatory cytokines [47]. In addition, Han et al. demonstrated that butyrate reduces pro-inflammatory cytokines interleukin (IL)-1β, IL-6, tumor necrosis factor (TNF)α, IL-8, and IL-12 and protects colon morphology against lipopolysaccharide (LPS)-induced colitis in weaning pigs [48]. Butyrate supplementation also alleviates diarrhea and upregulates tight-junction (TJ) proteins, such as claudin-3, occludin, and ZO-1, in weaning pigs [49]. Meanwhile, propionate has been reported to decrease serum and liver triglyceride levels and improve lipid metabolism in growing pigs [124]. Similar to butyrate, Zhang et al. reported that propionate increased jejunal expression of TJ proteins claudin-1, claudin-4, and occludin in pigs [50]. Propionate also promotes regulatory T cell (Treg) proliferation and regulates their function, resulting in colonic homeostasis [51]. Tregs play a crucial role in suppressing the immune response and their differentiation and potentiation are highly influenced by SCFA activation of G-protein-coupled receptor 43 (GPR43) [125–127]. Acetate, on the other hand, reduces inflammation by suppressing activation of inflammasomes via GPR43 [52]. The beneficial effects of SCFAs on intestinal health may also improve growth performance in pigs. In separate studies, Reyer et al. and McCormack et al. reported that pigs with high feed efficiency ([FE], leaner pigs) are associated with a higher abundance of SCFA-producing microbiota, such as Christensenellaceae, Oscillibacter, Cellulosilyticum, Rothia, Subdoligranulum, and Leeia [53,54]. Infusion of SCFAs in growing pigs resulted in higher feed intake and daily gain, as well as improved carcass and meat quality [55]. Furthermore, Gardiner et al. posited that microbiome-derived SCFAs promote insulin sensitivity and satiety in pigs, through GPR-activated stimulation of glucagon-like peptide-1 (GLP-1) and peptide YY secretion resulting in lower feed intake [6]. Meanwhile, lactate produced by lactic acid bacteria and Bifidobacteria helps to reduce the pH of the GIT, preventing the growth of potentially pathogenic bacteria, such as enterotoxigenic Escherichia coli [120].
BCFAs are produced through the deamination of the branched-chain amino acids valine, leucine, and isoleucine [60,62]. The BCFA concentration serves as a marker for protein fermentation, although it is also affected by the level of dietary carbohydrates [61,62,87,128]. In pigs fed with low dietary fiber, Heo et al. observed a decrease in the levels of BCFAs [129], while the addition of resistant starch also decreased BCFA levels [130]. Similar to butyrate, BCFAs can also be utilized by colonocytes as an energy source, although they are not preferred sources [56]. In previous studies, BCFAs were thought to have a protective function against inflammation in the premature intestine [57,58]. Moreover, BCFAs show a dose-dependent ameliorating effect against the pro-inflammatory cytokines TNFα and interferon (IFN)γ in pigs [59]. However, BCFAs are often associated with other byproducts of protein fermentation, such as ammonia and hydrogen sulfide, and are thus considered potentially harmful metabolites [60,131,132]. BCFAs can impair gut barrier function and enhance the expression of pro-inflammatory cytokines [60]. Nevertheless, additional evidence is required to determine the exact role of BCFAs in intestinal health.
Bile acids are hydroxylated amphipathic steroid acids produced from the liver via cholesterol metabolism [71,73]. In the normal state, bile acid plays an integral role in digestion, lipid absorption, and cholesterol and fat-soluble vitamins uptake [133]. Primary bile acids are produced from the conjugation of bile acids produced in the liver with taurine or glycine (also referred to as conjugated bile acids) [71,133]. The majority (95%) of primary bile acids are reabsorbed in the distal ileum via enterohepatic circulation. However, a small portion of these primary bile acids escapes, flows into the colon, and is metabolized by the microbiome into secondary bile acids [71,73,133]. Gut resident microbiota, such as Lactobacillus, Clostridium, Bacteroides, Bifidobacterium and Enterococcus can deconjugate taurine or glycine from the conjugated bile acids via bile salt hydrolase (BSH) enzyme to produce free primary acids in the colon [74,134]. These primary bile acids are then transformed into secondary bile acids such as deoxycholic acid (DCA) and lithocholic acid (LCA) via 7α-hydroxylation. Members of the Clostridium genus, such as Clostridium hiranonis, C. hylemonae, C. sordellii, and C. scindens, and genus Eubacterium are known to possess the bile acid-inducible (bai) operon, which is responsible for the 7α-hydroxylation activity of these genera [71–74]. There is a bidirectional interaction between bile acids and the gut microbiome – the gut microbiome metabolizing primary bile acids to produce secondary bile acids effectively alters the bile acid pool, while the secondary bile acids regulate the gut microbiome composition through their antimicrobial and cytotoxic activities [28,74,134,135].
According to recent studies, both primary and secondary bile acids interact with nuclear hormone receptors, specifically the Farnesoid X receptor (FXR) or G protein-coupled receptor (TGR5) [65,71]. This interaction is highly dependent on the affinity of each bile acid for the receptors [71]. Bile acid activation of TGR5 plays specific roles in glucose homeostasis (through the production of GLP-1), energy homeostasis, and inhibition of proinflammatory cytokines, whereas FXR activation contributes to the regulation of bile acid synthesis and lipid metabolism [65–67]. However, in dysbiosis, the normal interactions between bile acids and the gut microbiome are altered such that bile acids, especially secondary bile acids, can negatively impact swine intestinal health. Previous in vitro studies in porcine cells have demonstrated that excessive secondary bile acids, particularly LCA and DCA, decreased cell proliferation and gene expression of TJ proteins [68,69]. Moreover, Lin et al. showed that LCA downregulated the gene expression of catalase and superoxide dismutase, which are integral for relieving oxidative stress [69]. Although in vivo experiments focusing on swine bile acid profiles and microbiomes are limited, the results from these studies echo what has been observed in vitro. During a state of undernutrition in piglets, an increase in secondary bile acid concentration was observed, together with a decreased relative abundance of Lactobacillus [68]. Similarly, hyodeoxycholic acid (HDCA) feeding downregulated the expression of proliferating cell nuclear antigen (PCNA) and cyclin D1, markers of epithelial cell proliferation, in the ileum of weaning pigs, while enriching the population of known secondary bile acid producers such as Parabacteroides, Clostridia family XII TCG-001, and Lachnospiraceae [70]. These results suggested that bile acid metabolism in the gut microbiome is a potential target for improving swine health. However, the current data are still limited and thus require further investigation.
Polyamines are low-molecular-weight polycationic molecules produced by the gut microbiome through decarboxylation of aromatic or cationic amino acids [76,136]. Polyamines are important microbiome-derived metabolites, which play crucial roles in gene regulation, signal transduction, DNA and protein synthesis, protection against oxidative stress, and cell proliferation and differentiation [76,77,81,136]. Host colonocytes can produce polyamines (unlike SCFAs); however, the gut microbiome can also significantly contribute to the polyamine profile [87,88]. Putrescine, spermine, and spermidine are the main polyamines produced by the gut microbiome, whereas cadaverine, histamine, tyramine, and 5-aminovalerate are also produced in smaller amounts [77]. In the gut, numerous bacterial groups can metabolize amino acids into polyamines, including Clostridium XIVa group, Roseburia, and Ruminococcus [75]. Bacteroides and Fusobacterium also contribute to polyamine production in the large intestine [76,77]. Enterococcus, Streptococcus, Clostridium, Lactococcus, and Lactobacillus use anaerobic pathways to produce polyamines [77]. Bifidobacterium also has the capacity to produce polyamines [78]. Since these bacteria do not always have a complete set of enzymes to produce polyamines, the colonic microbiome is thought to work collectively to produce polyamines [138]. The polyamine concentration in pig intestines is highly influenced by the dietary protein levels [34,128,139]. Yu et al. reported that extremely low dietary protein levels result in lower tryptamine, cadaverine, and putrescine levels in pigs [140]. Similarly, Chen et al. demonstrated that a reduction in dietary protein from 18% to 12% reduces both ileal and colonic polyamine concentrations in growing pigs [141]. In contrast, a low protein diet enhances polyamine production. For instance, in a low-protein diet with antibiotic treatment, researchers have observed elevated polyamine concentrations in pigs [142,143]. In addition, Peng et al. reported that reduction of dietary crude protein to 15.3% increased total polyamines, especially cadaverine, but decreased upon further dietary protein reduction [144]. These contradictory results may be due to the presence of the amino acid lysine (precursor of cadaverine) even in the low-protein diet, as most researchers have noted in their respective reports.
To date, multiple studies have demonstrated the beneficial effects of polyamines on intestinal health in pigs. Polyamines (mainly putrescine, spermidine, and spermine) regulate swine gestation—they promote placental development, stimulate blastocyst formation, and inhibit apoptosis, potentially improving the survival of the fetus [80,81,145]. Polyamines are also crucial in the growth and maturation of the small intestine in early weaned piglets, as demonstrated by a series of studies [79,82–84]. Fang et al. reported that spermine enhances oxidative stress resistance in piglets [82]. Piglets fed spermine and spermidine-supplemented feed showed increased growth compared to those fed a normal diet, as reported by van Wettere et al. [83]. Lui et al. demonstrated that putrescine alleviates diarrhea and suppresses the expression of TNF-α, IL-6, and IL-8 in weaning piglets [146]. In contrast, Ewtushik et al. observed that polyamines caused detrimental damage to the intestinal morphology of early weaned piglets, which may be due to the concentrations and ratios of polyamines used in the experiment [85].
In addition to BCFAs and polyamines, microbial metabolism of amino acids also produces indolic and phenolic compounds [56,87,147]. These metabolites are produced by fermentation of tryptophan, tyrosine, and phenylalanine. Indole and indoleacetate are produced from the microbial fermentation of tryptophan, whereas tyrosine fermentation yields phenol, p-cresol, and 4-ethylphenol [87,88,94,147]. While phenylalanine fermentation produces phenylacetate and phenylpropionate [94]. High concentrations of indolic and phenolic compounds are produced in the distal colon, where the pH is near neutral and is favorable for the fermentation process to occur [56,87,147]. Amino acid fermentation is highly affected by dietary protein content; as such, elevated concentrations of indole and phenol have been observed in pigs fed a high-protein diet [56,62,132]. Anaerobic bacteria in the colon, such as Bacteroides, Clostridium, Peptostreptococcus, Bifidobacterium, Lactobacillus, and Escherichia are known to be involved in amino acid fermentation [86–88]. For instance, Clostridium sporogenes and Clostridium botulinum can produce indolepropionic acid through tryptophan metabolism [89]. Similarly, Peptostreptococcus contains phenyllactic acid dehydratase, which is responsible for the production of indole-3-acrylate [90]. Lactobacillus produces indole-3-aldehyde and indoleacetic acid from tryptophan [86]. Bifidobacterium can ferment tryptophan into indolelactic acid. Indolic compounds produced by the microbial fermentation of amino acids serve important functions in maintaining colonic health. Indolic compounds are important in stimulating the gut mucosal barrier through the activation of aryl hydrocarbon receptors (AhR) in intestinal epithelial cells [91,92] and upregulating the expression of TJ proteins such as claudin. Indole and its derivatives, such as indolepropionic acid, also reduce inflammation by activating the pregnane X receptor (PXR) [92,148]. In contrast, phenol and its derivatives exert deleterious effects on intestinal homeostasis. Phenol increases gut permeability, whereas p-cresol disrupts colonic cell respiration and ATP synthesis [60,93,94].
Among aromatic amino acids, tryptophan metabolism is perhaps the most studied in relation to pigs because the by-products of tryptophan fermentation, such as indolepropionic acid and indoleacetic acid, confer beneficial health effects [148]. In weaned piglets, increased colonic levels of microbiome-produced indole and indoleacetic acid coincided with the upregulation of AhR and increased TJ proteins ZO-1 and occludin [95]. Liang et al. reported that L-tryptophan supplementation improved the intestinal mucosal barrier of weaned pigs by upregulating ZO-1, ZO-3, and claudin-1, which were linked to the increased population of tryptophan-fermenting commensal bacteria Clostridium and Lactobacillus [150]. Indole and its derivatives also attenuate oxidative stress in diquat-treated pigs, as reported by Fu et al. [96]. On the other hand, skatole, like indole, is also a product of microbial fermentation of tryptophan and is associated with the occurrence of undesirable odor and meat taste called ‘boar-taint’ in barrows [97,98]. In their review, Wesoly and Weiler identified Clostridium and Bacteroides as the main commensal bacteria responsible for skatole formation [97]. Pieper et al. reported that skatole, together with tyrosine-derived products phenol and p-cresol, was elevated by high-protein diets [99]. They also noted that p-cresol negatively affected the growth performance of pigs [99].
Ammonia and hydrogen sulfide are also produced during the microbial fermentation of amino acids in the distal colon [87,103]. Bacterial production of ammonia involves either deamination of amino acids or hydrolysis of urea through ureases [56,88,132]. Several studies have focused on the role of Helicobacter pylori and H. suis in the production of ammonia during glutamine deamination in both humans and pigs [100,101]. Ammonia is considered a toxic fermentation by-product; thus, it is converted back to urea via enterohepatic circulation and excreted in urine [132]. In pigs, as well as in other hosts, a high-protein diet increases the production of ammonia; conversely, decreasing the protein or including fiber in the diet reduces ammonia production [56,103,151,152]. In a high-protein diet, where ammonia production is elevated, ammonia inhibits mitochondrial oxygen consumption and SCFA oxidation [87]. In addition, ammonia impedes the intestinal uptake of butyrate by downregulating monocarboxylate transporter 1 (a transporter protein) [56], which may explain the impaired protective effect of butyrate during increased ammonia levels [60]. However, the deleterious effect of ammonia is dose-dependent and usually requires higher concentrations to cause negative effects, as pointed out by Gilbert et al. [56]. Fermentation of methionine and cysteine, on the other hand, produces hydrogen sulfide [87,88,102]. Fusobacterium, Desulfovibrio, Escherichia, Salmonella, Clostridium, and Enterobacter have been reported to produce hydrogen sulfide from sulfur-containing amino acids [88,102]. These bacteria possess desulfhydrases that utilize these amino acids for energy [87]. Like ammonia, the effect of hydrogen sulfide on the host depends on its concentration. Hydrogen sulfide acts as an energy substrate for colonocytes and a signaling molecule at low micromolar levels; in contrast, at low millimolar levels, it inhibits cytochrome oxidase activity in the mitochondria [102,103]. At high concentrations, hydrogen sulfide can also hinder cell proliferation and induce intestinal inflammation [102]. The effects of gut microbiome-produced ammonia and hydrogen sulfide on the growth performance and intestinal health of pigs are not yet fully understood and require further investigation.
Neurotransmitters are important molecules in the central nervous system as they carry signals that control behavior and cognition [17]. These molecules can be either excitatory (glutamate, dopamine, and acetylcholine) or inhibitory (γ-aminobutyric acid [GABA], norepinephrine, and serotonin). In pigs, environmental stress factors (such as handling, housing, and weaning) have been known to affect the levels of neurotransmitters [153–155]. The gut microbiome regulates the gut–brain axis by producing these neurotransmitters or their precursors [17,25,26,104]. Some gut microorganisms, such as E. coli, Pseudomonas, and other known pathogens, also utilize these neuroactive metabolites as energy sources [104,110]. Strandwitz summarized most neurotransmitter-producing bacteria, such as Bifidobacterium and Lactobacillus (GABA), Bacillus and Escherichia (dopamine), Escherichia, Klebsiella, and Lactobacillus (serotonin) [104]. Biosynthesis of serotonin (5-hydroxytryptamine [5-HT]) by enterochromaffin cells is highly regulated by gut microbiome, specifically spore-forming bacteria [156,157]. Lactic acid bacteria are also known to produce trace amines (neuromodulating metabolites), including tyramine and tryptamine [17,26]. Notably, microbiome-produced neurotransmitters cannot cross the blood–brain barrier; thus, they act locally on the enteric nervous system or vagus nerves (in the case of GABA) or enteric dopaminergic neurons (in the case of dopamine) [17,104,158]. On the other hand, precursors of these neurotransmitters can cross the blood–brain barrier and influence the biosynthesis of neurotransmitters in the brain. The SCFA acetate traverses the blood–brain barrier into the hypothalamus and affects the biosynthesis of glutamate and GABA in the host [17]. Gut microbiome dysbiosis can lead to altered microbial production of neurotransmitters and their precursors, thus affecting behavior and cognition in pigs. In antibiotic-treated pigs, modulation of colonic microbiome leads to a significant reduction in neurotransmitters [159]. There is also significant evidence that altered gut microbiome and tryptophan-serotonin metabolism are associated with tail biting in pigs [105]. In contrast, dietary supplementation with tryptophan has been shown to alter the bacterial production of serotonin [106,107]. These studies emphasize the role of gut microbiome and diet in the biosynthesis of neurotransmitter chemicals, which ultimately affects neurological function in pigs.
Similar to the human gut microbiome, the pig gut microbiome can also synthesize vitamins [6,159,160]. Members of the genus Bacteroides are the main producers of water-soluble vitamin B, including riboflavin, thiamine, biotin, cobalamin, and folates [160,161]. Some species of Lactobacillus also have the capacity to de novo synthesize vitamin B [108,109]. B vitamins serve as coenzymes in neurological processes [110]. Vitamin K, an essential vitamin for proper blood clotting, is produced by the gut microbiome as well [108,110]. These gut microbiome-derived vitamins support the functional metabolism not only of the hosts, but also of other non-vitamin producing bacteria, such as Faecalibacterium, thereby extending their influence on intestinal health [161,162]. Our knowledge of the extent of the effects of gut microbiome-synthesized vitamins on pigs is still scarce and must be investigated in the future.
INFLUENCE OF PROBIOTICS SUPPLEMENTATION ON MICROBIAL METABOLITES
Probiotics, as defined by the FAO/WHO, are live microorganisms that, when administered in adequate amounts, confer health benefits to the host [13,164]. Since the ban on the use of antibiotics as animal growth promoters, the use of probiotics in livestock production has been steadily on the rise [12,13]. In swine production, the most common probiotic species used are Lactobacillus, Bacillus, Clostridium, Bifidobacterium, Enterococcus, and Saccharomyces [16,164-166]. To date, there is abundant evidence demonstrating the beneficial effects of probiotics on swine health and growth. Probiotic supplementation in pigs has been shown to improve average daily weight gain (ADG) and FE, stimulate the immune system, protect the host from pathogen invasion, improve nutrient digestion, and modulate gut microbiome [12,16,164,165,167,168]. The role of probiotics in swine maternal health, improving health in early life stages, and alleviating weaning stress in piglets has been highlighted in previous studies [169,170].
Probiotic supplementation affects gut microbiome composition and microbiome-derived metabolites (Fig. 1). Probiotics generally increase the concentrations of SCFAs, particularly acetate, butyrate, and propionate, in pigs [122,171–178]. Lactate levels can also be increased by probiotic supplementation due to the abundance of lactic acid bacteria [122,173]. The elevation in SCFA concentration was correlated with changes in the composition of the gut microbiome. Probiotic supplementation in pigs preferentially enriches the phylum Firmicutes, which consists of SCFA-producing bacterial groups, such as Lactobacillaceae, Ruminococcaceae, Erysipelotrichaceae, and Lachnospiraceae [122,171,175,178–180]. Furthermore, probiotic-mediated changes in the SCFA profile is associated with positive effects on the pigs, such as improved growth performance [171,172,174,175,178], improved intestinal morphology [176–178], stimulation of the host’s immunity [171,174], protection against pathogens [181], and improvement of cognitive performance [173]. The enhancing effect of probiotics on the SCFA profile of pigs is attributed to the increased ability of the gut microbiome to metabolize carbohydrates [182,183]. Probiotic-mediated elevation of Lactobacillus and Bifidobacterium in the GIT contributes to the elevation of SCFA levels, as these bacteria are known to generate SCFAs [16,161,183]. Lactate production also contributes to SCFA levels, specifically butyrate, through the cross-feeding mechanism of other commensals [112,184]. In contrast, studies on the effect of probiotics on the bile acid profile of pigs are currently limited. Nevertheless, data support the idea that probiotics can potentially alter the bile acid profile of pigs. Feeding of Lactobacillus plantarum to weaning piglets decreased the concentration of secondary bile acid secondary bile acid (LCA) in the ileum and increased the relative abundance of Lactobacillus while reducing the population of Clostridium and Parabacteroides [69]. Likewise, dietary supplementation with Lactobacillus delbrueckii proved to be efficient in lowering both primary and secondary bile acids in growing-finishing pigs [185]. Nealon et al. observed that Lactobacillus rhamnosus GG, combined with E. coli Nissle and rice bran, altered bile acid metabolism in pigs challenged with human rotavirus [186]. Additionally, the same authors highlighted the role of bile acids in the regulation of diarrhea, especially the secondary bile acid hyodeoxycholic acid (HDCA) [186]. The modulating effect of probiotics on the bile acid profile of swine may be attributed to the BSH activity of these probiotic strains, as well as their enriching effect on other gut resident microbiota with BSH activity, which promotes fecal excretion and neosynthesis of bile acids [135,185,187,188]. Another potential mechanism by which probiotics influence the bile acid profile is by reducing the population of gut clostridial species and other species known to produce secondary bile acids [69,178].
Microbial metabolites from amino acid fermentation (BCFAs, polyamines, indolic and phenolic compounds, and ammonia) are also influenced by probiotic feeding. Barba-Vidal et al. and Rodrigues-Sorrento et al. reported a reduction in colonic ammonia levels after supplementation with Bifidobacterium longum subsp. infantis in piglets challenged with ETEC K88 [189] or ETEC F4 [190] Meanwhile, probiotic feeding in pigs reduces skatole concentrations, as observed in multiple studies [177,180,191]. Similarly, supplementation of either Bacillus subtilis DSM 32315 with xylooligosaccharides (XOS) or a combination of Lactobacillus plantarum B90 and Saccharomyces cerevisiae P11 decreased indole concentrations [176,180]. Meanwhile, the effect of probiotics on polyamine levels is not always consistent, as observed in a series of studies [176,180,186], except for putrescine, which was consistently reduced by probiotic supplementation. Changes in amino acid fermentation metabolites always corresponds to changes in the composition of the gut microbiome; thus, modulation of the gut microbiome composition could be the main mechanism by which probiotics alter these metabolites [132,192]. Enrichment of beneficial bacteria, such Lactobacillus, Bacteroides, Bacillus, and SCFA-producing commensals, improves the enzymatic activities of digestive proteases and peptidases and increases the absorption ability of the epithelium [132,192]. In addition, probiotic consumption could offer protection from pathogenic and skatole-producing bacteria, such as E. coli through competitive exclusion or the production of antimicrobial peptides, such as bacteriocins [192]. Furthermore, probiotics potentially encourage the assimilation of ammonia into bacterial proteins, thereby reducing its excretion [132].
Probiotics can also be used to target the microbiome–gut–brain axis. Probiotics modulate the production of neurotransmitters in the gut, thereby modulating the signaling patterns in the gut–brain axis [193,194]. In rats, supplementation with L. rhamnosus JB-1 alters the levels of neurotransmitters such as GABA and glutamate, suggesting an underlying mechanism for the probiotic modulation of these chemicals. Currently, the use of probiotics to target the microbiome–gut–brain axis in pigs is limited. Based on the effects of probiotics on neurotransmitters and precursors in humans and other animal models, it is suspected that the effects might be similar in pigs. Indeed, Cao et al. reported that supplementation with Clostridium butyricum-based probiotics altered the levels of neurotransmitters (such as 5-HT, GABA, and dopamine) in weaned piglets [195]. Moreover, Bifidobacterium breve CECT8242 reverses the reducing effect of a high-fat diet on the neurotransmitter profiles of pigs [196]. A symbiotic preparation containing Lactobacillus salivarius and Lactobacillus reuteri improved the cognitive functions of pigs (although the authors did not measure changes in neurotransmitters in the study) [197]. Supporting investigations are still necessary to determine the potential of probiotics to affect the microbiome–gut–brain axis and their long-term effects on the pig production industry.
Notably, the beneficial effects of probiotics on the gut microbiome-derived metabolites are not consistently observed. For example, Nowak et al. did not observe any changes in the levels of indole, phenol, or p-cresol after supplementation with multispecies probiotics (Leuconostoc mesenteroides, Enterococcus faecium, and Carnobacterium divergens) [198]. Similarly, probiotic feeding did not change SCFA levels, as observed by Zhang et al. with Clostridium butyricum supplementation [199]. Conversely, changes in the microbiome and their metabolites may not always correspond to improvements in growth performance, FE, and other performance indices, as observed in multiple studies [122,173,191]. The inconsistencies in the observations of different studies may be attributed to several factors, including, but not limited to, the type of probiotics used (strain/species, single/multiple species), dose, age of pigs (neonatal, weaning, or finishing), duration of supplementation, other dietary components (fibers, protein concentration), husbandry practices, and varying environmental factors [16,167]. In addition to the potential reasons for the inconsistencies in reports, Ohashi and Ushida also noted that the effect of probiotics is significantly affected by the response of the indigenous gut microbiome [183]. Therefore, a careful approach must be exercised when selecting probiotics for pig production. A vast knowledge of the underlying mechanism of probiotics on swine gut microbiome and their metabolites is necessary to anticipate their overall impact on pig performance and health.
CONCLUSIONS
The significance of gut microbiome in modulating host health cannot be denied. Gut microbiome-derived metabolites, such as SCFAs, BCFAs, secondary bile acids, polyamines, indolic and phenolic compounds, and various neurotransmitters play crucial roles in maintaining and improving swine health. The effect of these metabolites can be beneficial or, in the state of dysbiosis or stress, deleterious to pigs. Thus, targeting the gut microbiome to modulate their metabolites is a key strategy for improving the growth of livestock animals. The dietary inclusion of probiotics can significantly alter the gut microbiome and the metabolites produced by it. Probiotics influence the production of metabolites by the gut microbiome. However, the effects of probiotics depend on many factors, and the results may not be consistent, as highlighted in this review. A comprehensive assessment must be conducted before supplementation with specific probiotics or probiotic combinations. Moreover, there is still a gap in the existing knowledge regarding the degree of the effects of gut microbiome-derived metabolites on swine health. In vitro and in vivo investigations of the mechanistic actions of these metabolites are extremely valuable for understanding their overall effects on pig health and growth. In addition, multi-omics techniques may provide valuable information on the complex interactions among the gut microbiome, metabolites produced by it, and swine health.