Background
Cows are infected by numerous pathogens that affect their productivity and health.These pathogens include bacteria, protozoa, and viruses. Diseases caused by pathogens affect the profitability of rearing animals [1,2]. Pathogen-associated Molecular Patterns (PAMPs) are recognized by pathogen recognition receptors (PRRs) on host cells to trigger immune responses [3]. Lipopolysaccharide (LPS), peptidoglycan (PGN), double-stranded viral RNA (Poly I:C) and bacterial oligonucleotides (CpG ODNs) are associated with infectious and metabolic diseases in the cow [2,4–6]. PAMPs mediate the production of cytokines by primarily binding to PRRs, such as TLRs on immune cells to activate several signaling pathways [7–9]. There are 10 TLRs identified in cows that recognize PAMPs [10]. Previous studies have reported that the increase of LPS in the digestive tract of the cow phenomenon called subacute ruminal acidosis, and its translocation into the bloodstream, results in immune responses which if not controlled, causes serious health consequences [11]. Proper recognition and elimination is essential to animal health and production [12]. Aside from TLRs, other receptors and co-receptors play significant roles in recognition and pathogen elimination.
Numerous studies have shown that Gal, a family of proteins defined by their affinity for β-galactosides, participate in the regulation of both innate and adaptive immunity [13–15].
Some galectins have been studied to bind microorganism-specific glycans, or host-like glycans on microorganisms to initiate immune responses [16]. Galectin mediated immune response could either lead to clearance of microorganism or favor establishment of the pathogens. Galectins (Gal) act as PRRs that orchestrate immune responses according to the level of pathogenicity of the invading microorganisms [17]. Some members of the galectin family are also known alarmins for sepsis, a condition caused by an overwhelming immune response to microbial infection [18].
The most significant advancement in animal health in the past three decades has been the paradigm shift from treatment of clinical illness to disease prevention [19]. Overuse of antibiotics in animal production has raised food safety and public health concerns in addition to its reduced effectiveness and resistance in animals [20]. As such, numerous studies are emerging to better understand the molecular mechanisms leading to infectious diseases to design more effective management practices to reduce cow health disorders [7,8]. An increased understanding of the genetics underlying the immune response mechanism of cows during infections may offer opportunities for alternative control strategies.
Because galectins are involved in immune responses and the outcome of microbial infections, it was essential to evaluate whether its expression in cows is affected by pathogen-associated molecular patterns (PAMP) stimulation in whole blood. Understanding galectin expression in response to PAMP stimulation will aid in drug design aimed at galectins as targets. This study aimed to evaluate the effect of stimulation with known PAMPs (natural and synthetic) on galectin gene expression in cow blood.
Materials and Methods
The Institutional Animal Care and Use Committee of North Carolina A&T State University approved all protocols for animals handling. Five multiparous Holstein Friesian periparturient cows (N = 5) from North Carolina A&T State Dairy farm were used. None of the animals showed any signs of disease or received medications during the 4-week period before blood sampling.
Complete details of blood sampling have been reported elsewhere [21]. Briefly, Whole blood (10 mL) was collected aseptically from the jugular vein of the animals into vacutainer tubes containing 1mL of the anti-coagulant Acid Citrate Dextrose. The tubes were gently mixed and placed on ice immediately after collection. The samples were transported to the Laboratory for Animal Genomic Diversity and Biotechnology at North Carolina A&T State University for further analysis.
One mL of blood from the cows was each incubated with 10 μg/mL each of either Escherichia coli derived LPS (Sigma-Aldrich, St. Louis, MO), Staphylococcus aureus-derived PGN (Sigma-Aldrich), CpG ODN (2216) class A (Invivogen, San Diego CA ), CpG ODN (2006) class B (Invivogen) and 12.5 μg/mL of poly I:C (Invivogen) individually to assess the expression of LGALS. Samples treated with phosphate buffer saline (PBS) served as control. Samples were incubated at 37°C, with 85% humidity and 5% CO2 for 30 minutes. At the end of the incubation period, the tubes were spun down at 1,700 × g at 4°C for 10 minutes. Supernatants were collected and stored at –80°C to measure secreted galectins concentration. Trizol (1 mL) was added to cell pellets and stored for RNA isolation (Ambion®, Thermo Fisher Scientific Inc, Waltham, MA).
Total RNA was isolated using Trizol according to the manufacturer’s instructions (Sigma-Aldrich St. Louis, MO). The appropriate precautions were used to avoid RNase contamination throughout the entire procedure [9]. The RNA concentration (ng/μL) and purity (260/280) were assessed using a Nanodrop Spectrophotometer ND 1000 (Thermo Scientific Inc., Waltham, MA). Total RNA was pipetted into an RNA 6000 Nano LabChip® (Agilent Technologies, Santa Clara, CA) and RNA integrity was determined using Bioanalyzer following manufacturer’s protocol (Agilent). Complimentary DNA (cDNA) synthesis was performed with 500 ng/μL RNA (purity 260/280 = 1.8, RIN = 7). Retroscript kits (Ambion®) were used to synthesize cDNA for real-time Polymerase chain reaction. cDNA synthesis was performed in an MWG AG Biotech Primus 96 Plus Industrial Lab Thermal Cycler (MWG Biotech Huntsville, AL). Total RNA (1 μg) was heated to 85°C for 3 minutes and placed on ice, centrifuged briefly, and returned to ice. The remaining RETROscript® components were added: 10X RT (PCR) buffer (2 μL), dNTP mix (4 μL), RNase inhibitor (1 μL), and MMLV-RT (1 μL), for a final volume of 20 μL. The components were mixed gently, centrifuged briefly and incubated at 42–44°C for 1 hour, followed by an incubation step at 92°C for 10 minutes to inactivate the Reverse Transcriptase (RT). Polymerase chain reaction was performed by assembling the following components: RT reaction buffer (5 μL), 10X PCR buffer (5 μL), dNTP (2.5 μL), Nuclease-free water (37.5 μL), PCR primers (provided in kit) (2.5 μL), and thermostable DNA Polymerase (1–2 units (U)). The PCR cycle was: Step 1) Initial denaturation, 94°C for 2 minutes; Step 2) Amplification, 94°C for 30 seconds (denaturation), 60°C (annealing) for 30 seconds, 72°C (extension) for 1 minute; Step 3) to Step 2 for 30 cycles; and Step 4) Final Extension, 72°C for 5 minutes.
With the use of Primer-3 online tool (http://bioinfo.ut.ee/primer3-0.4.0/), Forward and reverse primers for cow galectin genes LGALS1, 2, 3, 4, 7, 8, 9, 12, and 15 were designed commercially. Based on published sequences, cow specific Glyceraldehyde 3-phosphate dehydrogenase (GAPDH) and β actin were purchased from MWG, Biotech Huntsville AL. β-actin and GAPDH were used as internal controls and for normalization.
Real-time qPCR was performed in the CFX Connect real-time system (Bio-rad Laboratories, Hercules, CA). The qPCR reaction mixture consisted of template, primer (μL), intercalating dye SYBR Green, DH2O, 200 ng of cDNA, 1 μL (100 μM) of forward primer, 1 μL (100 μM) of reverse primer, 10 μL of SYBR Green and DH2O to the volume of 20 μL.
All samples were carried out in triplicate 20-μL reactions in 96-well plates, and a negative control with no cDNA template were included in every run. The run program used were: Step 1) 95°C for 15 seconds for denaturing, Step 2) 60°C for 30 seconds for primer annealing, and 72°C for elongation. Step 3) to Step 2 for 30 cycles; and Step 4) Final Extension, 72°C for 5 minutes. Specificity of the amplicon products was confirmed by visual inspection of melting curves. Real-time PCR data was analyzed using the Livak’s method [22]. The housekeeping genes (GAPDH and β actin) and samples from far-off/PBS treated cows were used to determine the ΔΔCt [22]. Fold change in transcript abundance was calculated using the Livak method. Where
Commercial bovine specific galectin ELISA kits were purchased and used to determine the concentrations of secreted Gal-1 (catalog no. MBS2882620, detection range; 0.31 ng/mL & 20.0 ng/mL), Gal-2 (catalog no. MBS033680 detection range; 0.625 ng/mL & 20 ng/mL), Gal-3 (catalog no. MBS017323 detection range; 0.156 ng/mL & 10 ng/mL), Gal-4 (catalog no. MBS028694, detection range; 0.25 ng/mL & 8 ng/mL), Gal-8 (catalog no. MBS041856 detection range; 0.5 ng/mL & 16 ng/mL), Gal-9 (catalog no. MBS033074 detection range; 0.625 ng/mL & 20 ng/mL), Gal-12 (catalog no. MBS032400, detection range; 31.2 pg/mL & 1,000 pg/mL) in plasma according to the manufac-turer’s instructions (My BioSource®)) [23]. A microplate reader was used to measure the absorbance at 450 nm (BioTek Instruments, Inc., Winooski, VT). Galectin concentration was then determined using a standard curve. The sensitivity was 0.1 ng/mL for all assays. Both intraassay CV (%) and inter-assay CV (%) for all assays was less than 15%.[CV (%) = SD/mean ×100].
Real-time PCR data were analyzed using Livak’s method [22]. Housekeeping genes (GAPDH and β actin) and samples from PBS treated samples were used to determine the ΔΔCt, as described above. The Proc GLM procedure in SAS 9.4 (SAS Institute Inc, Cary NC) was used to analyze data obtained for total galectins concentrations. Each sample was assayed in triplicates. The PDIFF statement in SAS (SAS Institute Inc. Cary, NC) was used to compare all least square means. Significant differences were declared at p < 0.05. PROC corr was used to do a correlation analysis between LGALS and Gal concentrations in plasma.
Results
LPS increased transcription of LGALS4 and LGALS12 (2.5 and 2.02 folds respectively) (Fig. 1A). PGN increased transcription of LGALS-1, -2, -3, -4, -7, and -12 (3.0, 2.3, 2.0, 4.1, 3.3, 2.4 folds respectively) (Fig. 1b). Poly I:C increased the transcription of LGALS1, LGALS4, and LGALS8 (1.78, 1.88, and 1.73 folds respectively) (Fig. 1c). CpG oligodeoxynucleotides 2006 (CpG ODN2006) did not cause any significant fold changes in LGALS transcription (FC < 2) (Fig. 1d). CpG oligodeoxynucleotides 2216 (CpG ODN2216) increased transcription of LGALS1 and LGALS3 (3.8 and 1.6 respectively), but reduced LGALS2, LGALS4, LGALS 7, and LGALS12 (–1.9, –2.0, –2.0 and –2.7) (Fig. 2).
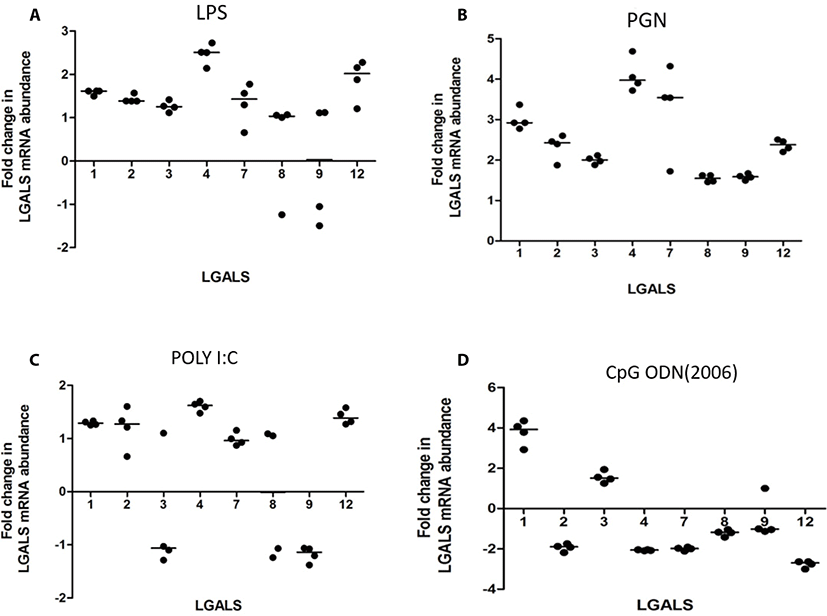
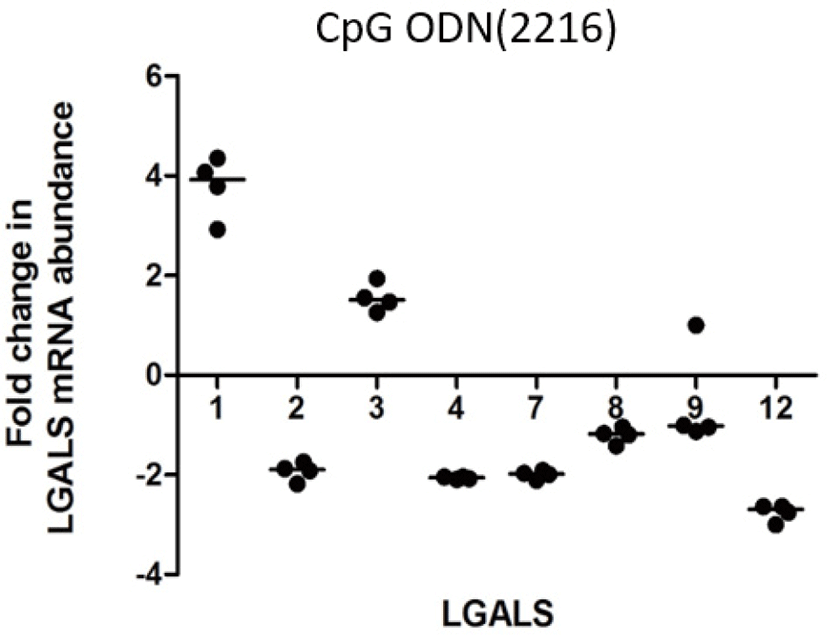
Poly I:C and CpG ODN2006 increased plasma secretion of Gal-1 compared to control (PBS) (p = 0.10 and p = 0.0125 respectively) (Fig. 3a). CpG ODN 2216 increased Gal-2 concentration in plasma compared to control (PBS) (p = 0.0459) (Fig. 3b). CpG ODN 2216 and CpG ODN 2006 increased Gal-3 compared to control (PBS) (p = 0.013 and p = 0.0195 respectively) (Fig. 3c). LPS, CpG ODN2216, and CpG ODN2006 reduced Gal-4 concentrations in plasma compared to control (PBS) (p = 0.04, p = 0.0005 and p = 0.0273 respectively (Fig. 4a). PGN and Poly I:C increased Gal-8 secretion compared to control (PBS) (p = 0.001 and p <.0001 respectively (Fig. 4a). PGN and Poly I:C increased Gal-9 secretion compared to control (PBS) (p = 0.001 and p <.0001 respectively) (Fig. 4b). PAMPs did not affect plasma concentration of Gal-12 (p > 0.05) (Fig. 4c).
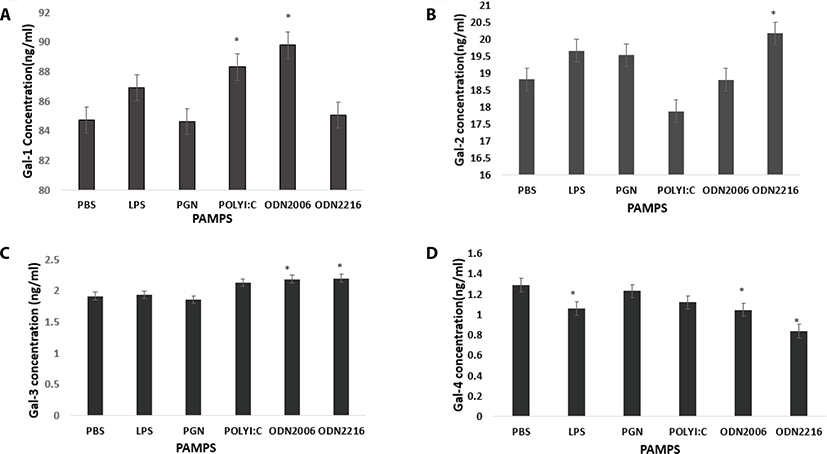
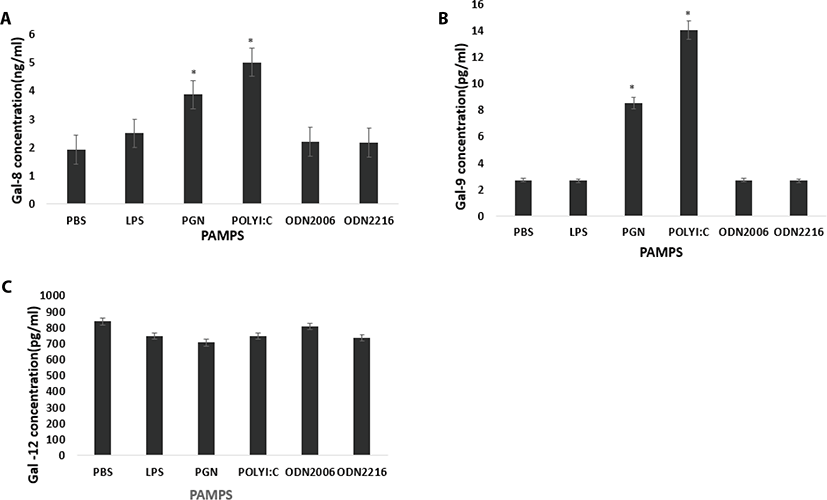
LGALS1 correlated negatively with Gal-1 (p = 0.02) and correlated positively with Gal-2 (p = 0.03). LGALS2 correlated positively with Gal-4 (p =< 0.0001) and Gal-3 (p = 0.0009). LGALS3 correlated negatively with Gal-1 (p = 0.006) and Gal-3 (p = 0.05). LGALS4 correlated positively with Gal-4 (p =< 0.0001) and negatively with Gal-3. LGALS8 correlated positively with Gal-8 (p =< 0.0001), Gal-9 (p =< 0.0001) and Gal-4 (p = 0.0002), and negatively with Gal-2 (p =< 0.0002). LGALS9 correlated positively with Gal-9 (p = 0.007), Gal-8 (p = 0.0006) and Gal-4 (p = 0.002), and negatively with Gal-3.
LGALS12 correlated positively with Gal-4 (p =< 0.0001) and negatively with Gal3 (p = 0.0073).
Discussion
In the past few years, galectins have been shown to participate in the regulation of both innate and adaptive immunity [13,24]. Furthermore, there is research that supports the ability of Gals to recognize microbial pathogens like viruses, bacteria and protozoan parasites [15] directly. The ability of Gals to function as PRRs in the immune defense against invading microbes makes them indispensable components in the innate immune response [16]. To test whether microbial infection affects galectin expression in cow blood, we treated blood with natural bacteria cell wall components (LPS and PGN), synthetic bacterial DNA adjuvants (CpGODN2006 and CpGODN2216) and viral RNA (Poly I:C). Treatment of cells with PAMPs mimic microbial infection and regulates expression of various genes [25]. The current study showed that PAMP modulates LGALS gene transcription as well as Gal secretion differentially in cow blood. Both transcription and secretion depended on the type of stimulant used.
Bacterial LPS and PGN designated PAMPs, are recognized by TLR-4 and TLR-2 respectively [26]. The binding of these PAMPs to their receptors is characterized by transcription of genes involved in immune responses and secretion of cytokines [8,9,27]. In the present study, LPS stimulation increased the transcription of LGALS4 and LGALS12 in cow blood (Fig. 1a). Gal-4 has been studied to be only expressed in inflamed cells and therefore has a more restricted distribution in normal cells [28]. Gal-4 also promotes resolution of inflammatory diseases, therefore, making it an important player in immune responses [29]. Gal-12, on the other hand, is preferentially expressed in adipose tissues and has also been studied to be associated with impaired metabolic conditions [30]. Both Gal-4 and Gal-12 exert regulatory functions in immune cells and have strong potential as biomarkers. This data suggests a possible role of Gal-4 and -12 in the recognition and resolution of gram-negative bacteria in cows. The relationship between LPS and the transcription of these galectins need to be explored.
The gram-positive bacterial cell wall, PGN also increased transcription of LGALS4 and LGALS12 as well as all galectins tested. The differential LGALS transcription and secretion in response to LPS and PGN observed in the study suggest distinct immunological activities in response to these PAMPs. This also accentuates the fact that both gram-positive and gram-negative are recognized by different receptors to elicit different immunological responses. Thus, either LPS or PGN can be used as adjuvants to induce the production of different galectins.
Recently, galectins have been observed to interact directly with the β-galactosides on the surface of viruses thereby participating in antiviral defense, via the activation of the innate and adaptive immune responses [31,32]. Previous research has shown that viral infection has modulatory effects on galectin expression and function [33]. In this study, galectin expression in blood in response to viral challenge assessed by a PolyI:C challenge. Poly I:C is a viral PAMP that promotes cellular recognition of RNA viruses by binding to TLR3 to induce proinflammatory, as well as regulatory and cytokine re-sponses [34]. In this study, Poly I:C increased the transcription of LGALS1, LGALS4, and LGALS8 with a subsequent secretion of Gal-1, -3, -8 and 9 in plasma. In a previous study, secretion of several galectins, including Gal-1 and -3 were increased in virus-infected macrophages [35]. Gonzalez et al. (2005) has also postulated that induced Gal-1 secretion after a viral infection is beneficial for the virus due to its anti-inflammatory functions [31]. On the contrary, Gal-3 has opposing functions since it recruits inflammatory cells to the site of infection. It is possible that the balance between the secreted extracellular Gal-1 and -3 in the current may regulate the direction of the inflammatory response in cows. It is also noteworthy that Gal-8, which has been known to be involved in phagocytosis [36] is transcribed and secreted upon viral PAMP stimulation. The current study suggests that these galectins may also be involved in recognition of viral PAMPs and promote the removal of these viral pathogens through phagocytosis, or prevent viruses from entering the host cell.
Studies have indicated that oligodeoxynucleotides (CpG ODNs) containing unmethylated CpG dinucleotides are potent activators of both innate and adaptive immunity [37]. CpG ODNs are recognized by the Toll-like receptor 9 (TLR9) [38]. The TLR9 signaling pathway involves mitogen-activated protein kinases, and NF-kB-inducing kinase-IKK- Inhibitor of KappaB Kinase (IkB) pathways [39]. Both CpG ODN 2216 and CpG ODN 2006 have been studied to show distinct functional profiles depending on the type of CD8+ cells they are exposed to. CpG ODN 2216 induces high amounts of interferon alpha in plasmacytoid dendritic cells whereas CpG ODN2006 only induces small amounts of IFN alpha [40]. Furthermore, CpG ODN2006 has also been studied to be a weak activator of natural killer cells compared to CpG ODN2216 [41]. This proves that both CpG ODN motifs show distinct immunologic activities. In the present study, the 2 types of CpG ODN adjuvants also affected LGALS transcription and secretion distinctively in cow blood. Although no fold changes were observed in LGALS transcription (FC < 2), CpG ODN2006 treatment increased secretion of Gal-1 and Gal3 in plasma (Fig. 1d). This increase supports the proinflammatory roles of CpG ODNs since Gal-1 and Gal-3 are proinflammatory in pathogenic conditions. CpG ODN2216, however, increased Gal-2, a pro-apoptotic galectin and Gal-3, a proinflammatory galectin. This also points to a balance in immune response upon PAMP recognition. Improper orchestration of the immune response to microbial infections may lead to sepsis, a condition caused by an overwhelming immune responses [7].
It was also important to note from this study that although LPS and the CpG ODNs motifs are both of bacteria origin, LPS did not affect galectin secretion in plasma. This could mean that Gal secretion in cow blood is increased in response to bacteria DNA compared to its cell wall components. The concentration of LPS (10 μg) used as well as the length of exposure could also cause variation in transcription and translation in blood [42]. Also, the fact that transcription of LGALS4 was reduced in blood treated the CpG ODN motifs is noteworthy since both PAMPs are synthetic adju-vants. This points to a negative regulatory effect of these PAMPs on LGALS4. The results of this study have impli-cations for vaccination and use of synthetic PAMPs in cows and immunomodulation.
Conclusion
The results demonstrate that PAMPs differentially modulate transcription of mRNA and secretion of Gal in cow blood. Elucidation of the relationship between PAMPS and galectin expression may help to define their roles in infectious diseases as well as aid in drug design for the dairy industry.