INTRODUCTION
Enteric CH4 is considered not only an indicator of gross energy losses, but also a potent greenhouse gas with a global warming potential that is 28 times higher than that of carbon dioxide (CO2) [1,2]. Enteric CH4 is produced by methanogenic archaea through methanogenesis following one of three pathways: hydrogenotrophic, methylotrophic, or acetoclastic [3–5]. In light of this, several dietary mitigation strategies have been conducted to reduce enteric CH4 emissions from ruminants [5,6]; however, little is known about the role of mineral supplementations in achieving this. Li et al. [7] reported decreased CH4 emissions and methanogen populations in lactating cows following dietary mineral salt supplementation. They explained that dietary mineral salt reduced CH4 emission through the reduction of methanogen phenotypes and the A : P ratio, while increasing H+ ion utilization in propionate production. However, that study did not focus on the effects of minerals on heat-stressed dairy cattle or steers.
Ruminant production is greatly hampered by adverse environmental conditions, especially heat stress (HS) [8-10]. HS reduces dry matter intake (DMI), which subsequently hampers energy and protein metabolism, leading to increased metabolic disorder, mineral imbalance, and several other health problems [11-14]. The sensitivity and response to HS varies among breeds [15,16]. Holstein steers have been reported to exhibit a significant reduction in DMI under HS [16]. Additionally, studies have found that both Jersey steers and Jersey dairy cows are less sensitive to HS than Holstein steers [15,16]. Therefore, both Holstein and Jersey steers were considered in this study. Furthermore, the influence of HS on rumen microbial alteration and enteric methane (CH4) emissions in Holstein and Jersey steers has already been reported [16]. During HS, cattle require more supplementation with trace minerals in their diet to prevent the adverse effects of HS [17]. Some trace minerals, such as zinc (Zn), copper (Cu), and selenium (Se), have been used in diets to minimize the adverse effects of HS in ruminants because of their antioxidant effects [14,18–23]. However, organic trace minerals have a more beneficial effect than inorganic minerals on ruminants [18]. Therefore, organic trace minerals were considered in this study. Although the National Research Council (NRC) [24] has recommended the dose of these minerals under normal conditions, the optimal dose required to overcome the adverse effects of HS during summer has not yet been established. Hence, during HS, we supplied minerals at a level of 70% based on the maximum tolerable concentration recommended by the NRC [24]. It has been previously reported that different trace minerals have toxic effects on methanogens. Hernández-Sánchez et al. [25] reported that Cu decreases CH4 production because it is toxic to some rumen methanogens. Liu et al. [26] further reported that mineral supplementation reduces enteric methane emissions by altering the rumen microbiome. Dietary Se improves rumen fermentation by altering the rumen microbiome; however, it also has antimicrobial effects that may affect rumen methanogen diversity [27]. Therefore, this study evaluated the effects of supplementation with organic forms of Zn, Cu, and Se minerals on enteric CH4 emissions, rumen fermentation characteristics, rumen bacteria, and methanogens in heat-stressed Holstein and Jersey steers.
MATERIALS AND METHODS
The study was conducted at the Sunchon National University (SCNU) animal farm and the ruminant nutrition and anaerobic laboratory in the Department of Animal Science and Technology, SCNU, Suncheon, Korea. All animals used in this study and all experimental protocols were reviewed and approved by the Institutional Animal Care and Use Committee (approval number: SCNU-IACUC-2020-06).
Three non-cannulated Holstein steers (710.33 ± 43.02 kg; approximately 30 months old) and three Jersey steers (559.67 ± 32.72 kg; approximately 30 months old), were kept in two separate areas with a 3×3 Latin square design. For both experiments, the diets included a Control group (Con) fed the basal total mixed rations (TMR) without mineral supplementation, an NRC recommended concentration of mineral supplementation group (NM) fed TMR with (Se 0.1 ppm + Zn 30 ppm + Cu 10 ppm)/kg dry matter (DM), and a higher concentration of mineral supplementation group (HM) fed the basal TMR with (Se 3.5 ppm + Zn 350 ppm + Cu 28 ppm)/kg DM. Organic form of Se (Yeast-Selenium; X-SEL 3000TM, Algebra Bio, New South Wales, Australia), Zn-glycinate (BASF SE, Ludwigshafen, Germany), and Cu-glycinate (BASF SE) were used to supplement Se, Zn, and Cu, respectively.
The duration of the feeding experiment for each period was 20 d. This comprised diet adaptation and reaming for the first 14 days and CH4 measurement and rumen fluid sampling for the following 6 days. A 7 d washing period was maintained between each Latin square. The ingredients and chemical composition of the basal TMR are presented in Table 1. Individual stalls with feeding and water facilities were available for the experimental steers. The Con group was fed only basal TMR once a day at 09:00 h with a refusal rate of 5%–10%, whereas the respective concentrations of minerals were mixed well with the basal TMR and fed to the NM and HM groups. The DMI was measured as the difference between the feed offered and feed refused. Basal TMR was collected twice (on days 7 and 14) during the experiment. Dry matter content was estimated using a hot-air oven at 65°C for 72 h [28]. Proximate analysis of TMR was performed using standard methods [29]. The protocols of Van Soest et al. [30] and Van Soest [31] were used to determine the neutral detergent fiber (NDF) and acid detergent fiber (ADF) content, respectively. This study was conducted under the condition of HS with an average temperature-humidity index (THI) of 82.79 ± 1.10, which was calculated as THI = (0.8 × ambient temperature) + [% relative humidity/100 × (ambient temperature − 14.4)] + 46.4 [32].
Enteric CH4 emissions were measured using an automated head chamber system (AHCS) or GreenFeed (GF) unit (C-Lock, Rapid City, SD, USA), as described by Hristov et al. [33] with slight modifications. Briefly, all steers were trained to familiarize themselves with the GF unit before the experiment started to avoid any sort of psychological stress. To measure CH4 emissions, each steer visited the GF at eight different time points within three consecutive days in each measurement period. The 0 h or before feeding at 9 am, 9 h after feeding (6 pm), and 18 h after feeding (3 am) time points were considered for CH4 measurement on day 1, while 3 h after feeding (12 pm), 12 h after feeding (9 pm), and 21 h after feeding (6 am) were considered on day 2, and 6 h after feeding (3 pm) and 15 h after feeding (12 am) were considered on day 3. The GF unit was installed in one corner of a large pen, and at each measurement time point, all steers were successively moved to this pen from their individual stalls. To attract the animals to the GF unit and ensure a proper head-down position within the hood at the time of measurement, molasses-coated concentrated pellets (250–300 g/visit) were used. The number of pellets ingested per steer per day was excluded from DMI calculation. All relevant data of animal entry and exit times to the GF unit, standard gas calibration information, CO2 recovery time point, and amount of gas release data were sent to C-Lock. The calculated CH4 production (g/d) data were obtained using a web-based data management system, and the CH4 yield (g/kg DMI) was calculated.
In each period, rumen fluid was collected from each steer on the last day of the feeding trial, before the morning meal, using a stomach tube. The first 300 mL of rumen fluid was discarded to avoid contamination of rumen fluid by saliva. Ruminal pH was measured immediately after collection using a pH meter (SevenCompactTM pH/Ion meter S220, Mettler Toledo, Greifensee, Switzerland). Along the sides, three aliquots were prepared separately from each rumen fluid and transported to the laboratory in the presence of dry ice. These samples were stored at -80°C for further analysis of ammonia nitrogen (NH3-N), volatile fatty acids (VFA), and rumen microbiota. The rectal temperature (RT) of the steers was also recorded at approximately 12 pm on the same day of sampling using a digital thermometer (WPT-1, CAS, Yangju, Korea).
NH3-N concentration was measured using a Libra S22 spectrophotometer (CB40FJ, Biochrom, Cambourne, UK) according to the protocol described by Chaney and Marbach [34]. VFA concentration was measured using high-performance liquid chromatography (HPLC; Agilent Technologies 1200 series, Agilent Technologies, Waldbronn, Germany) according to the protocol described by Han et al. [35]. To perform HPLC, a UV detector (set at 210 nm and 220 nm), METACARB87H column (Varian, Palo Alto, CA, USA), and buffered solvent (0.0085 N H2SO4; at a flow rate of 0.6 mL/min) were used.
For DNA extraction and subsequent metataxonomic analysis of rumen microbiota, all rumen fluid samples were sent to Macrogen (Seoul, Korea). Briefly, DNA from rumen fluid was extracted using a DNeasy PowerSoil Kit (Qiagen, Hilden, Germany), according to the manufacturer’s protocol [36]. The quality and quantity of DNA were assessed using PicoGreen and NanoDrop, respectively. To prepare amplicon libraries of each sample for both bacteria and archaea, two separate sequence runs were performed with two different primer sets. In order to prepare the amplicon library of each sample for bacteria, the Illumina 16S Metagenomic Sequencing Library protocols were used which was performed using two-step polymerase chain reaction (PCR) amplification of the 16S rRNA genes with the primers Bakt_341F (5′-TCGTCGGCAGCGTCAGATGTGTATAAGAGACAGCCTACGGGNGGCWGCAG-3′) and Bakt_805R (5′-GTCTCGTGGGCTCGGAGATGTGTATAAGAGAC AGGACTACHVGGGTATCTAATCC-3′) of the V3–V4 region at an annealing temperature of 55°C [37]. For archaea, 787-F (5′-TCGTCGGCAGCGTCAGAT GTGTATAAGAGACAGATTAGATACCCSBGTAGTCC-3′) and 1059-R (5′- GTCTCGTGGGCTCGGAGATGTGTATAAGAGACAGGCCATGCACCWCCTCT -3′) primer sets of–V5-V6 were used, with an annealing temperature of 63°C. AMPure beads (Agencourt Bioscience, Beverly, MA, USA) were used to purify the products of the first and second PCR. The individual amplicon libraries were normalized after quantification using PicoGreen. They were then size verified using a TapeStation DNA ScreenTape D1000 (Agilent Technologies), pooled at an equimolar ratio, and sequenced on a MiSeq system (Illumina, San Diego, CA, USA) using a 2 × 300 bp kit. After sequencing, Illumina MiSeq raw data were classified by sample using an index sequence, and a paired-end FASTQ file was generated for each sample. The sequencing adapter sequence and F/R primer sequence of the target gene region were removed using Cutadapt (v3.2) [38].
For error correction of the amplicon sequencing process, the DADA2 (v1.18.0) [39] package of the R (v4.0.3) program was used. For paired-end reads of bacterial sequences, the forward sequence (Read1) and reverse sequence (Read2) were cut to 250 bp and 200 bp, respectively, and sequences with expected errors of two or more were excluded. However, 200 bp and 150 bp were considered for the same archaeal sequences. An error model for each batch was then established to remove noise from each sample. After assembling the paired-end sequence corrected for sequencing error into one sequence, the chimera sequence was removed using the DADA2 consensus method to form amplicon sequence variants (ASVs). In addition, for the comparative analysis of the microbial community, the QIIME (v1.9) [40] program was used for normalization by applying subsampling based on the number of reads of the sample with the minimum number of reads among all samples.
For each ASVs sequence, BLAST+ (v2.9.0) [41] was performed in the Reference DB (NCBI 16S Microbial DB for bacteria and NCBI NT DB for archaea), and taxonomic information for the organism of the subject with the highest similarity was assigned. At this time, if the query coverage of the best-hit matching the DB is less than 85% or the identity of the matched area is less than 85%, the taxonomy information is not allocated. A comparative analysis of various microbial communities was performed using QIIME with the above ASVs abundance and taxonomic information. The Shannon index and inverse Simpson index were obtained to check the species diversity and uniformity of the microbial community in the sample, and the alpha diversity information was confirmed using the rarefaction curve and Chao1 value. Based on the weighted and unweighted UniFrac distances, beta diversity between samples (information on microbial community diversity among samples in the comparison group) was obtained, and the relationship between samples was visualized using Principal Coordinates Analysis (PCoA) [40].
All data for DMI, CH4 emissions, rumen fermentation, and rumen microbiome were analyzed using the mixed procedure of SAS. Here, we considered breed and trace minerals as factors. Then, we tested whether there were any breed differences using a general linear model along with Duncan’s multiple range test. All analyses were performed using SAS (version 9.4, SAS Institute, Cary, NC, USA) [42]. Statistical significance was set at p < 0.05.
RESULTS
The DMI and enteric CH4 emissions of Holstein and Jersey steers with varying mineral supplementation levels are presented in Table 2. In both breeds, DMI (kg/d) did not differ significantly among the treatment groups (p > 0.05). CH4 production (g/d) and CH4 yield (g/kg DMI) were significantly higher in Jersey steers than in Holstein steers (p < 0.05); however, a numerical decrease in CH4 production and yield was observed with increasing concentrations of mineral supplementation in both breeds (p > 0.05). The highest RT was recorded in the Con group, followed by the NM and HM groups in both breeds; however, the differences were not statistically significant (p > 0.05). The rumen fermentation characteristics of Holstein and Jersey steers supplemented with different levels of minerals are presented in Table 3. Ruminal pH was significantly higher in Holstein steers than that in Jersey steers (p < 0.05); however, a similar pH was observed among treatment groups (p > 0.05). The NH3-N concentration (mg/dL) was similar between breeds (p > 0.05); however, it was influenced by trace minerals and the interaction between breeds and trace minerals (p < 0.05). In Holstein steers, the Con group had the lowest NH3-N concentration, whereas the HM group had the highest NH3-N concentration when compared with Jersey steer groups (p < 0.05). The total VFA concentration (mmol/L) was higher in Holstein steers than in Jersey steers (p < 0.05); however, no differences were observed in the concentrations of total VFA or molar proportions of propionate and butyrate among different mineral supplemented groups in both breeds (p > 0.05). Although trace mineral supplementation had a significant influence on the molar proportion of acetate, regardless of breed (p < 0.05), no significant differences were observed between groups in each breed (p > 0.05).
Con, only TMR (without mineral supplementation); NM, TMR + NRC recommended concentration of mineral supplementation (Se 0.1 ppm + Zn 30 ppm + Cu 10 ppm)/kg DM; HM, TMR + higher than recommended concentration of mineral supplementation (Se 3.5 ppm + Zn 350 ppm + Cu 28 ppm)/kg DM; B, breed effect; T, trace mineral supplementation effect; B × T, interaction effect between breed and trace mineral supplementation.
Con, only TMR (without mineral supplementation); NM, TMR + NRC recommended concentration of mineral supplementation (Se 0.1 ppm + Zn 30 ppm + Cu 10 ppm)/kg DM; HM, TMR + higher than recommended concentration of mineral supplementation (Se 3.5 ppm + Zn 350 ppm + Cu 28 ppm)/kg DM; B, breed effect; T, trace mineral supplementation effect; B × T, interaction effect between breed and trace mineral supplementation.
a-c Means with different superscripts in a row differ significantly among different treatment groups in Holstein steers while
A total of 531,527 bacterial and 1,326,280 archaeal quality-filtered sequence reads were obtained from sequencing 18 rumen fluid samples. The average sequences obtained from each sample for bacteria and archaea were greater than 29,500 and 73,600, respectively. Good’s coverage was greater than 99% for each sample. The Holstein steers showed tentatively higher ASV and Chao1 richness estimates (p = 0.051 and 0,052, respectively) and significantly higher Shannon and inverse Simpson diversity indices compared to Jersey steers, regardless of trace mineral supplementation (p < 0.05) (Table 4). Rarefaction measures for rumen bacteria are presented in Fig. 1. The beta diversity data were not significant between breeds or among the trace mineral supplementation groups (Fig. 2). However, the above-mentioned parameters were not influenced by trace mineral supplementation regardless of breed or the interaction between breeds and trace mineral supplementation (p > 0.05) (Table 4). The relative abundance of most bacterial phyla was not influenced by breed, trace mineral supplementation, or the interaction between them (p > 0.05). Jersey steers had a higher relative abundance of the phylum Candidatus Melainabacteria than Holstein steers (p < 0.05); however, no differences were observed among the different trace mineral supplementation groups in either breed (p > 0.05). In Holstein steers, at the phylum level, Bacteroidetes (accounting for 58.82% to 74.78%) and Firmicutes (19.07% to 36.79%) were the two major bacterial taxa among all treatment groups (Fig. 3, Table 5). Likewise, Bacteroidetes (which ranged from 66.21% to 69.63%) and Firmicutes (23.83% to 26.62%) were the top two bacterial phyla among the treated Jersey steers (Fig. 3). At the genus level, most of the more abundant bacterial genera were not influenced by breed, trace mineral supplementation, or the interaction between them (p > 0.05); however, the genus Capnocytophaga was more abundant in Jersey steers than in Holstein steers, regardless of trace mineral supplementation (p < 0.05) (Fig. 4; Table 6).
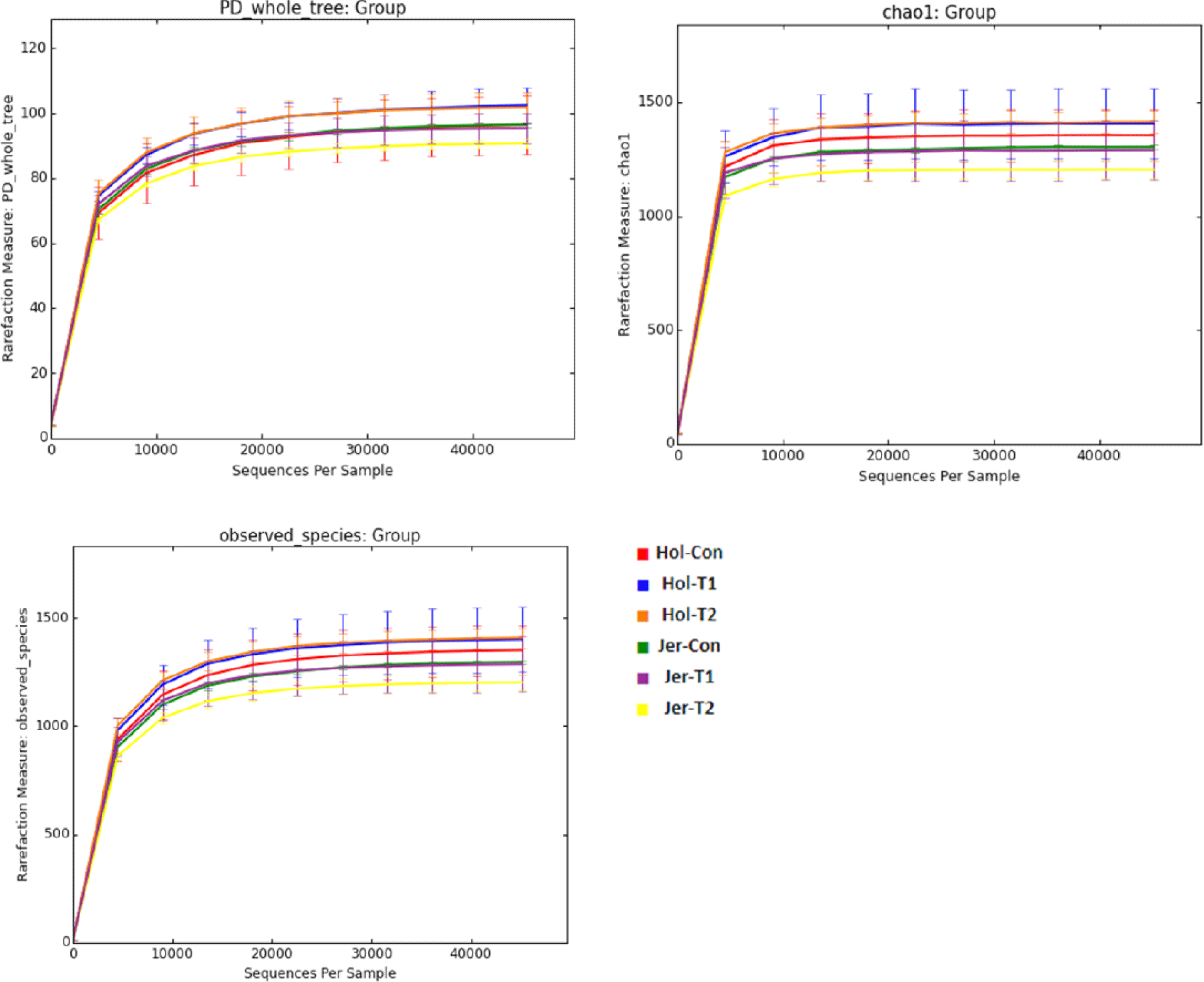
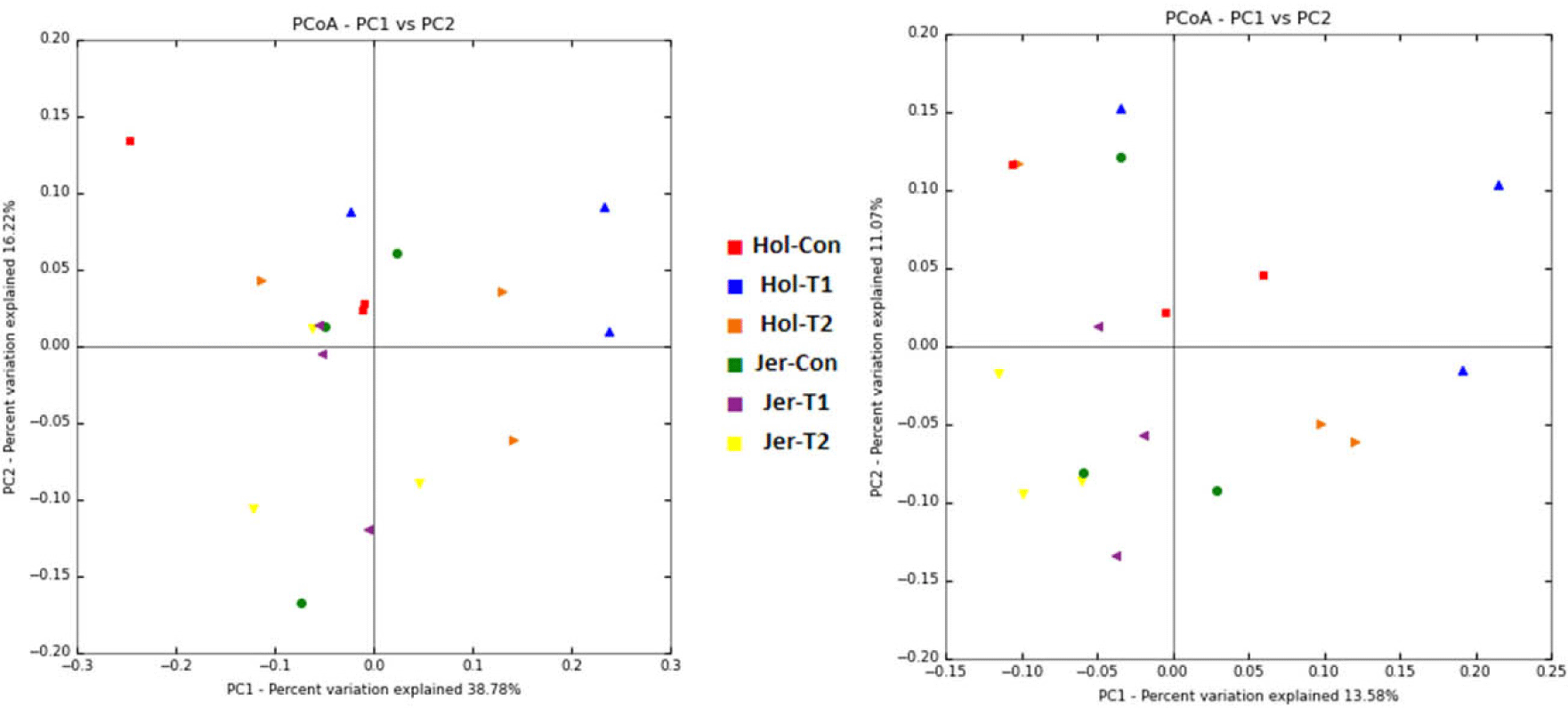
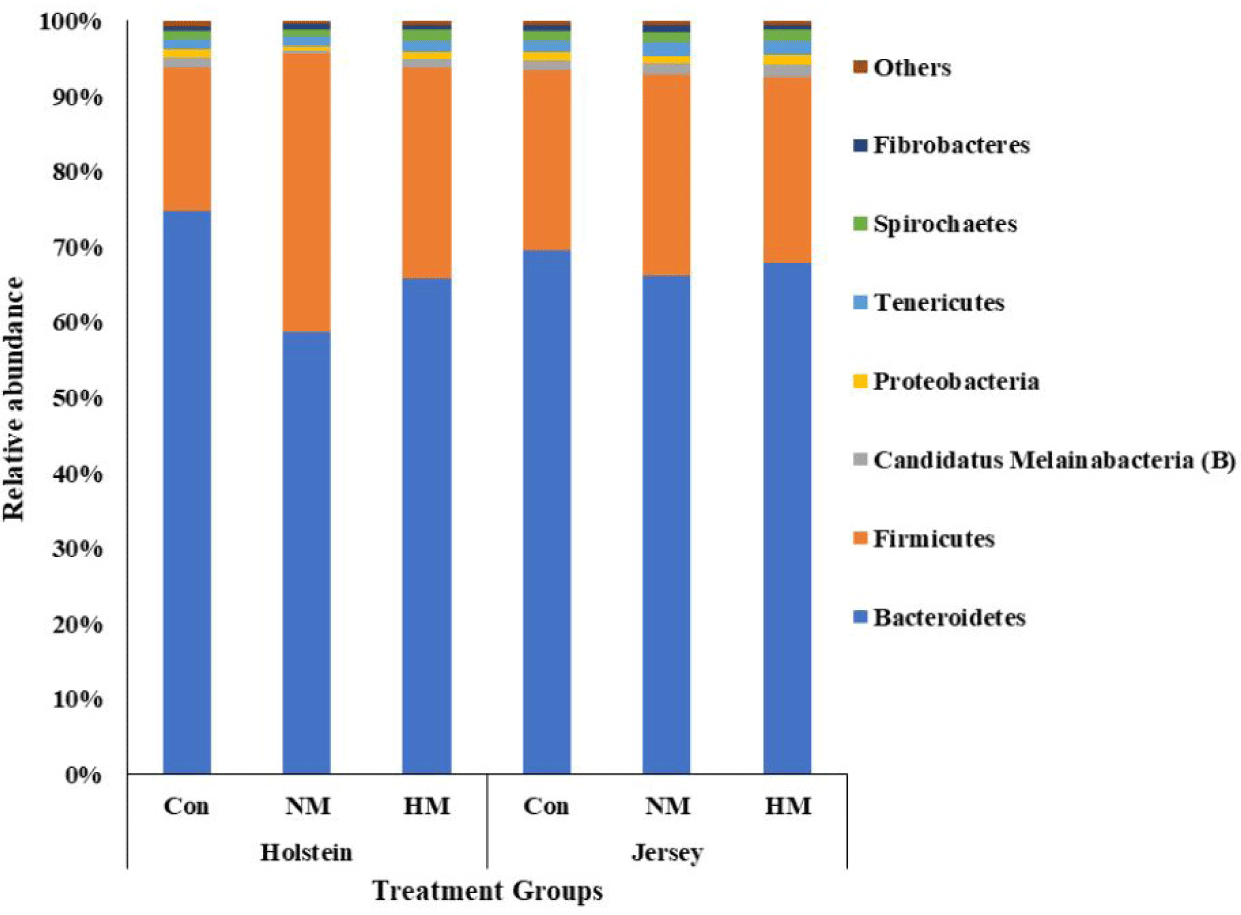
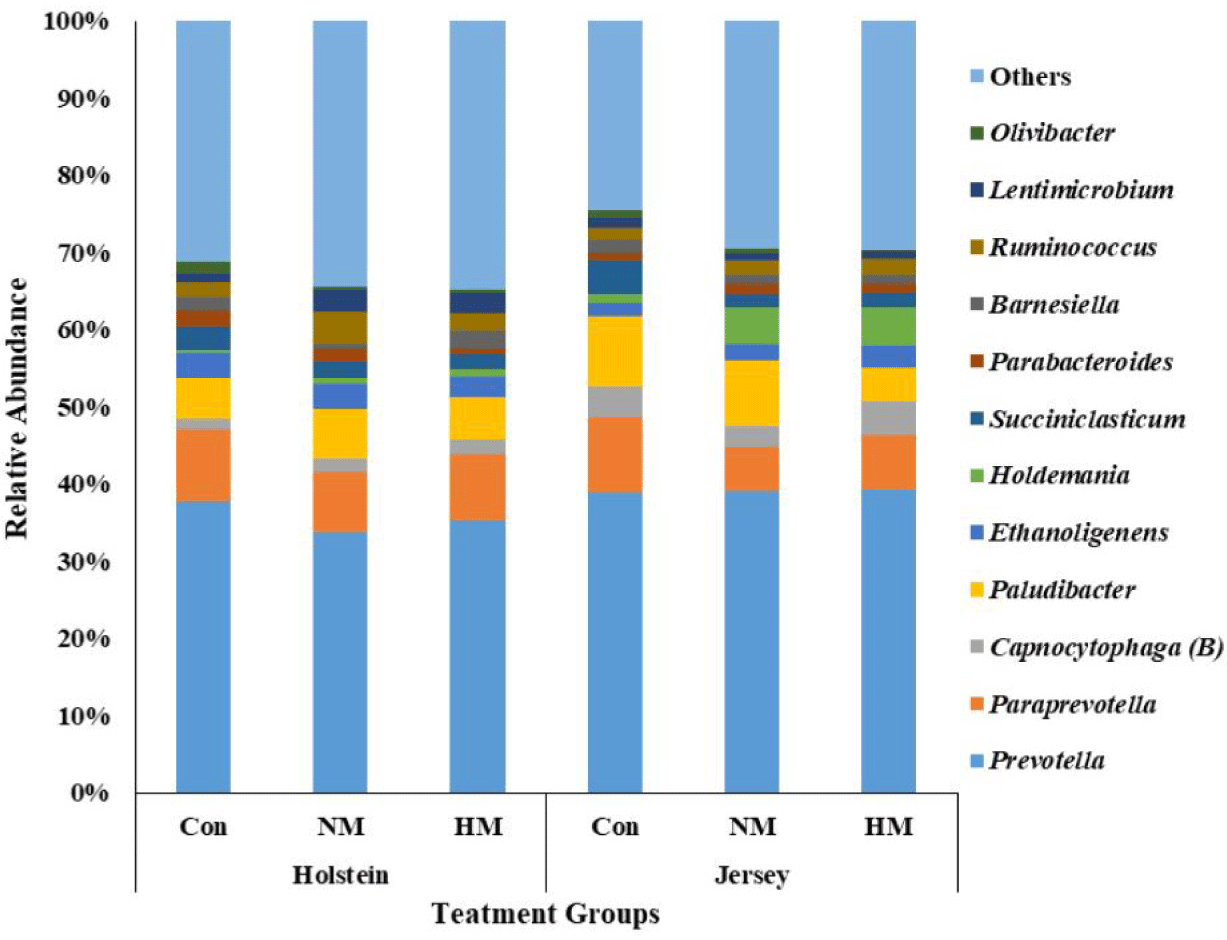
Con, only TMR (without mineral supplementation); NM, TMR + NRC recommended concentration of mineral supplementation (Se 0.1 ppm + Zn 30 ppm + Cu 10 ppm)/kg DM; HM, TMR + higher than recommended concentration of mineral supplementation (Se 3.5 ppm + Zn 350 ppm + Cu 28 ppm)/kg DM; B, breed effect; T, trace mineral supplementation effect; B × T, interaction effect between breed and trace mineral supplementation.
Methanobrevibacter (ranging from 57.30% to 72.99% in Holstein steers and from 58.17% to 82.04% in Jersey steers) was the most abundant methanogen among all treatment groups for both breeds (Fig. 5A; Table 7). The relative abundance of Methanobrevibacter was not influenced by breed and trace mineral supplementation (p > 0.05) but was tentatively influenced by the interaction between breed and trace mineral supplementation (p = 0.065). Methanomassiliicoccus (15.74% to 34.57% in Holstein and 14.20% to 37.75% in Jersey steers) was the second most abundant methanogen among all treatment groups for both breeds. The relative abundance of Methanomassiliicoccus was not influenced by breed (p > 0.05); however, it was tentatively influenced by trace mineral supplementation (p = 0.061) and the interaction between breed and trace mineral supplementation (p = 0.072). Jersey steers showed a tendency toward decreasing Methanobrevibacter abundance and increasing Methanomassiliicoccus abundance with higher concentrations of trace mineral supplements (p = 0.072 and 0.086, respectively). However, in Holstein steers, only the lowest abundance of Methanobrevibacter and highest abundance of Methanomassiliicoccus were recorded in the HM group (p > 0.05). Among the remaining genera, Methanosarcina and Methanobacterium were more abundant, while Methanomicrobium was less abundant in the Holstein steers than in Jersey steers, regardless of the trace mineral supplementation (p < 0.05); the relative abundance of these methanogens was not influenced by trace mineral supplementation or the interaction between breed and trace mineral supplementation (p > 0.05). At the species level, the methanogens Methanobrevibacter thaueri, Mbr. olleyae, Mbr. millerae, and Methanomassiliicoccus luminyensis were the top four methanogen species among all the treatment groups in both breeds (Fig. 5B, Table 8). Among them, Mbr. olleyae were more abundant in Holstein steers than in Jersey steers, regardless of trace mineral supplementation (p < 0.05). Relative abundance of Mma. luminyensis was tentatively influenced by trace mineral supplementation and the interaction between breed and trace mineral supplementation (p = 0.061 and 0.072, respectively). A tendency toward an increasing pattern of Mma. luminyensis abundance was observed in Jersey steers with increasing mineral concentration (p = 0.086). Among the remaining methanogens, the Methanosarcina mazei, Mbr. oralis, and Methanobacterium aggregans were more abundant in Holstein steers, whereas the relative abundances of Mbr. boviskoreani, and Methanomicrobium mobile were greater in Jersey steers, regardless of trace mineral supplementation (p < 0.05).
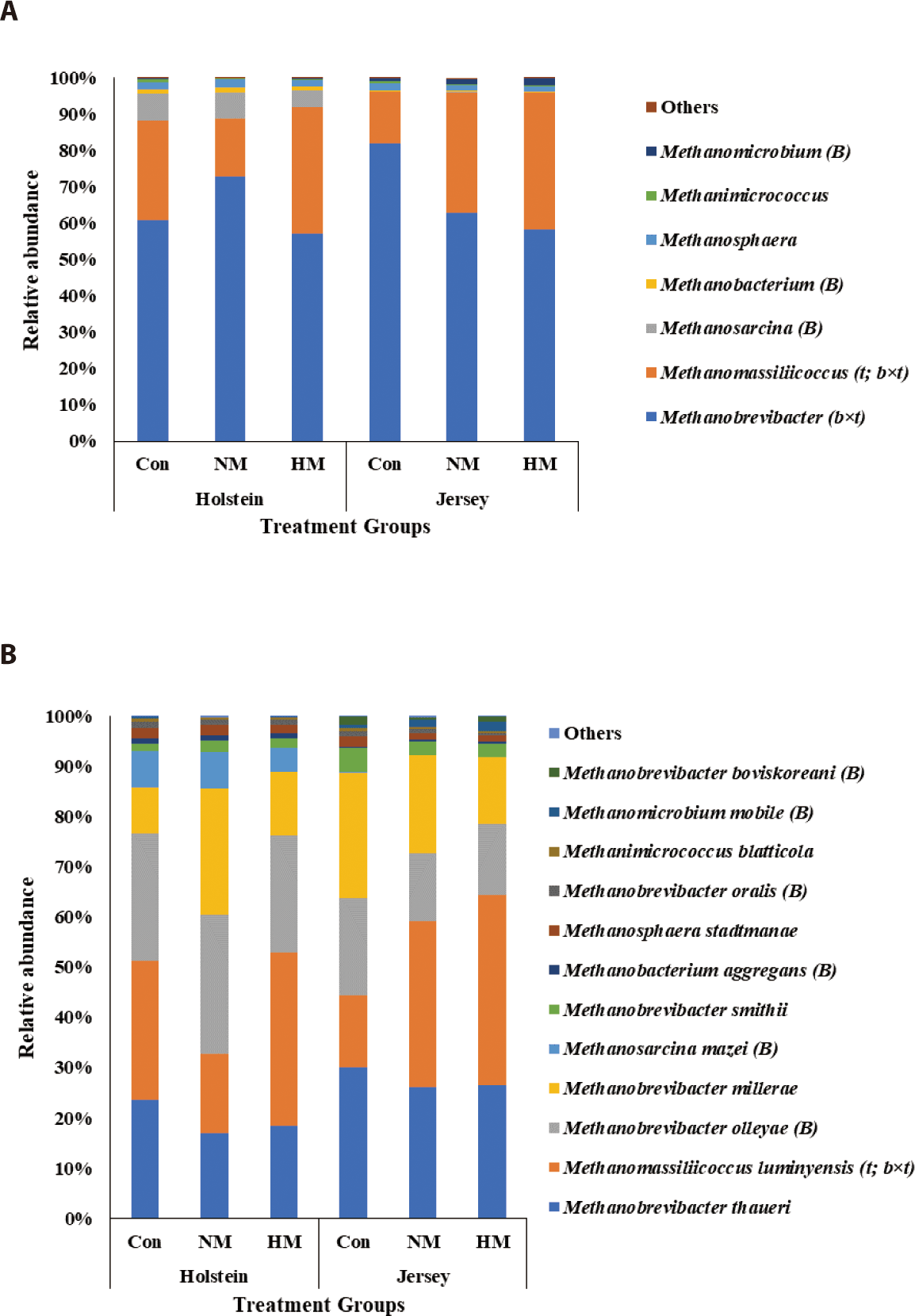
DISCUSSION
Dietary supplementation, particularly mineral supplementation, is one of the most important strategies used to reduce the adverse effects of HS in ruminants. However, determining the minerals to use, as well as their most effective concentrations, is a major challenge. Therefore, this study focused on using higher than the recommended concentrations of dietary mineral supplements (Zn, Cu, and Se) during HS. RT is considered a physiological parameter of HS. Although the RT did not vary significantly among different treatment groups in both breeds, the value confirmed that the animals were in HS, which was also observed in a study by Joo et al. [43]. HS reduces DMI, which affects ruminant performance [11,13]. However, similar DMI was observed among the different treatment groups in both breeds, indicating that, in this study, dietary trace minerals had no influence on DMI. These non-significant findings among the different treatment groups were expected because the present study was conducted under HS conditions, with high THI (82.79 ± 1.10); consequently, these findings cannot be compared with the findings expected under normal conditions.
VFA production is negatively correlated with ruminal pH [44]. In this study, the ruminal pH and total VFA were not influenced by trace mineral supplementation; however, lower ruminal pH was recorded in Jersey steers than in Holstein steers, which is in agreement with the findings of Islam et al. [45]. This might be due to the higher total VFA production by Jersey steers compared to that by Holstein steers in this study. Rumen NH3-N concentration depends on the status of dietary protein breakdown in the rumen, rumen microbial utilization, and ruminal epithelial absorption [46,47]. In this study, breed had no influence on the NH3-N concentration. Although the mineral-supplemented groups had higher NH3-N concentrations than the Con group in both breeds, the concentrations were within normal ranges.
The rumen microbiome helps break down the feed substrate in the rumen and improve animal performance. Bacteroidetes and Firmicutes are the major bacterial phyla in different breeds, including Holstein and Jersey breeds [16,27,48–52]. Likewise, in the present study, Bacteroidetes, followed by Firmicutes, were the two most abundant bacterial phyla among all the treatment groups in both breeds. Furthermore, a previous study reported that HS alters the rumen microbiota in Holstein and Jersey steers [16]. However, the similar relative abundance of major bacterial phyla and genera among the treatment groups for both breeds suggests that higher concentrations of mineral supplementation did not alter rumen bacterial community composition in the present study. The higher relative abundance of Capnocytophaga in Jersey steers suggests their preferential growth in Jersey steers compared with Holstein steers, which was supported by the findings of Islam et al. [45].
Methanogenesis is the process of CH4 production by methanogens in the rumen via two different pathways: hydrogenotrophic and methylotrophic [6]. Methanobrevibacter is the major archaeal genus involved in the hydrogenotrophic pathway, whereas Methanomassiliicoccus is involved in the methylotrophic pathway [3]. In this study, while the differences were not significant, Methanobrevibacter was the most abundant archaeal genus among all treatment groups in Holstein (collectively around 90%) and Jersey steers (collectively > 95%), followed by Methanomassiliicoccus. Methanosarcina is another identical methanogen that can produce CH4 via three different pathways, namely the hydrogenotrophic, methylotrophic, and acetoclastic pathways [3]. In this study, a significantly higher relative abundance of Methanosarcina was observed in Holstein steers than in Jersey steers, suggesting their preferential growth in the rumen of Holstein steers. The higher relative abundance of the methanogen species Mbr. olleyae was reported in Holstein steers than in Jersey steers. Similarly, King et al. [53] reported a higher relative abundance of Mbr. olleyae in Holstein cows which produced lower CH4 emissions than Jersey cows. They further reported that CH4 production was negatively correlated with the RO group containing Mbr. ruminantium and Mbr. olleyae and was positively correlated with the SGMT group (consisting of Mbr. smithi, Mbr. gottschalkii, Mbr. millerae, and Mbr. thaurei). The significantly lower CH4 production and yield observed in Holstein steers compared with that in Jersey steers in the present study further corroborates these findings. Dietary mineral salt supplementation has been reported to decrease CH4 emissions and methanogen population in lactating cows [7]. However, trace mineral supplementation had no influence on CH4 production, yield, and methanogen abundance regardless of breed, which may be due to the short-term supplementation of dietary trace minerals in this study.
CONCLUSION
Supplementation with high concentrations of dietary organic trace minerals (selenium, zinc, and copper) did not alter enteric CH4 emissions or the methanogenic community. However, Holstein steers emitted low enteric CH4, with a higher relative abundance of Mbr. olleyae than Jersey steers.