INTRODUCTION
Recently, the use of phytochemicals in meat-producing animals has gained importance due to their bioactive properties, including antioxidants, antimicrobials, immunomodulators, or growth promoters [1]. Ferulic acid (FA, 4-hydroxy-3-methoxycinnamic acid) is a phytochemical commonly linked to polysaccharides such as arabinoxylans, xyloglucans pectin, and cell walls, and is present in a wide range of natural sources, including fruits, vegetables, cereals, and grains [2,3].
Recent studies with FA supplemented in meat-producing animals have shown a reduction in fat thickness and improved carcass yields and meat quality in pigs, bovines, and lambs; however, the mode of action remains unclear, and several potential mechanisms have been suggested like mimicking β-AA action by increase of muscle protein synthesis and reduction of body fat, hormonal activation or antioxidant like preventing endogenous protein oxidation [47]. In contrast, β-adrenergic agonists (β-AA) are synthetic growth promoters, analogs of catecholamines, and are commonly used in animal production with significant results in growth performance and carcass traits [8]. The biological mechanism is through binding to the β-adrenergic receptor (β-AR) situated on the cell surface of skeletal muscle and adipose tissue [9]. These compounds generate an overexpression of β1-AR, β2-AR, and myosin heavy chain (MHC) and hormonal factors such as insulin growth factor (IGF), changes in protein synthesis rates, and larger muscle fibers [10,11].
β-AA compounds such as zilpaterol hydrochloride (ZH) have adverse effects on meat quality and are banned from the European Union or Asian countries [12,13]. Thus, the use of natural compounds such as FA can be a favorable alternative to synthetic growth promoters. Currently, there are no data on the histological and gene expression of lambs supplemented with FA. Supplementation of commercial lambs with FA (300 mg or 600 mg d−1) might improve the productive parameters and protein synthesis by β-AR binding and evidenced by MHC isoforms expression. The objective of the present study was to evaluate the growth performance, carcass traits, fiber characterization, and skeletal muscle gene expression in hair lambs supplemented with two doses of FA.
MATERIALS AND METHODS
Growth performance and carcass evaluations were conducted at an ovine commercial farm located in the northwestern region of Mexico (100° N, 27° W) during spring. Morphometry of muscle fibers was performed at the Centro de Investigación y de Estudios Avanzados, located in Ciudad de Mexico, Mexico. The gene expression evaluation was conducted at the Centro de Investigación en Alimentación y Desarrollo (CIAD), located in Hermosillo, Sonora, Mexico. All handling procedures involving lambs were performed within the guidelines of approved official techniques for animal care in Mexico (NOM-051-ZOO, 1995: Humanitarian care of animals during mobilization; NOM-033-SAG/ZOO, 2014: Slaughter methods for domestic and wild animals). All protocols and experimental procedures were approved and supervised by the Research Ethics Committee of CIAD (ID: CE/034/2018).
The study was performed with 32 male lambs (Dorper × Pelibuey) with an initial weight of 24 ± 3 kg at 4 months of age. All lambs were allocated to individual pens equipped with feeders and waterers. The lambs were adapted to the pens and a basal diet for two weeks prior to the start of the experimental period. The basal diet was formulated according to the recommendations of the National Research Council (Table 1). Prior to the experimental trial, lambs received an injection of vitamins A, D, and E (Vigantol, Bayer, Mexico City, Mexico; 1 mL/animal) and were treated for internal and external parasites (Ivermectin, Sanfer Laboratory, Mexico City, Mexico; 0.5 mL/animal). At the end of the adaptation period, the animals were individually weighed and randomly assigned to one of the following treatments (n = 8 per treatment): 1) a basal diet without additives (control); 2) 300 mg d−1 of FA (FA300; Laboratorios Minkab SA, de CV, Guadalajara, Jalisco, Mexico); 3) 600 mg d−1 of FA (FA600); and 4) 6 mg d−1 of ZH (Grofactor®, Laboratorio Virbac, Mexico). The feeding period was 32 days, and ZH was withdrawn 72 h prior to animal slaughter.
To ensure the complete intake of additives, initially, each dose of FA300, FA600 or ZH were weighed and immediately mixed with 50 g of the basal diet and given to each individual lamb, ensuring the total consumption of the additive with the 50 g of feed, later the rest of the diet without additive was provided in the feeders. In the control group, 50 g of diet without additives was also given. Fresh drinking water was available during the experiment, and feed was provided at 0800 h and 1600 h.
Climatic conditions at the experimental site ranged from 17°C to 37°C, and the average relative humidity (RH) was 30.5%. The temperature-humidity index (THI) was calculated according to the equation described by Hahn [14] with the temperature average (Te) and RH: THI = 0.81 × Te + RH / 100 (Te − 14.40) + 46.40. Based on the THI values, climatological conditions were considered thermoneutral (THI ≤ 74, Te = 20.68 ± 2.17°C, RH = 42 ± 9.58%, THI = 65.72 ± 2.55 units). The health status of the animals was monitored throughout the experiments.
The lambs were weighed individually to record their initial and final body weights. The average daily gain (ADG) was estimated as the difference between the initial and final weights divided by the feeding days. The feed provided and feed refused in each pen were recorded daily to calculate feed intake and expressed as dry matter. Feed conversion per pen/treatment based on the average feed intake and ADG was calculated.
All slaughter procedures complied with the current regulations (NOM-033-SAG/ZOO, 2014: Slaughter methods for domestic and wild animals). The lambs were fasted from feed and water for at least 12 h before slaughter. Subsequently, the lambs were transported to the abattoir located next to the commercial farm and slaughtered by exsanguination. The lambs were skinned and eviscerated, and the hot carcass weight (HCW), dressing percentage, Longissimus thoracis (LT) area in cm2, and fat thickness (mm) at the 12th rib from the left side of the carcass were recorded. Muscle pH was measured at 45 min and 24 h postmortem at the 12th intercostal space using a portable pH meter (HANNA HI 99163, Mettler Toledo Process Analytical. Wilmington, MA, USA).
LT muscle samples were collected at 10 min postmortem (transversal cuts at 10th intercostal space from the right side of the carcass) and immediately cryopreserved with cooled isopentane (Sigma-Aldrich, St. Louis, MO, USA) and liquid nitrogen for histoenzymatic and gene expression analysis and shipped to CIAD.
Transverse sections of cryopreserved muscle were cut to 10 µm thickness using a cryostat microtome at −20°C (CM-1100, Leica Microsystems, Nussloch, Germany) and mounted on glass slides for subsequent analysis of nicotine-amide adenine dinucleotide tetrazolium reductase (NADH-TR) and alkaline ATPase staining. Briefly, to determine the characteristics of oxidative or glycolytic fibers, muscle sections were stained using the NADH-TR technique [15]. Then, glass slides with muscle sections were incubated in Coplin staining jars for 1 h at 37°C in nitro‐blue tetrazolium (NBT)-NADH solution 1:1 v/v (1.2 mM NBT [Sigma-Aldrich] diluted in 50 mM Tris buffer [BioRad, Irvine, CA, USA], pH 7.6; 2.25 mM nicotinamide adenine dinucleotide [NADH, Sigma-Aldrich] diluted in 50 mM Tris buffer [BioRad]]. The glass slides were then washed three times with deionized water. Excess NBT-NADH solution was removed with acetone washes (30%, 60%, and 90%).
The alkaline ATPase technique (pH 9.4) was performed according to the method described by Guth and Samaha [16]. Muscle sections in glass slides were submerged in Coplin staining jars with pre-incubation solution (Tris base 10 mM [BioRad] and 18 mM CaCl2 [J.T. Baker, Mexico City, Mexico], pH 10.3) for 15 min. Subsequently, the glass slides were washed three times with deionized water and incubated for 1 h at 37°C in a working solution of Tris buffer with adenosine 5′-triphosphate (Sigma-Aldrich) at pH 9.4. Muscle sections were washed with 200 mM CaCl2 for 3 min, followed by 2% (w/v) CoCl2 (Sigma-Aldrich, Gillingham, UK) for 3 min and 10% (v/v) ammonium sulfide (Sigma-Aldrich) for 3 min, and then washing with deionized water.
Muscle fibers of both techniques were measured using a light optical microscope (Nikon Eclipse E600, Nikon, Tokyo, Japan) adapted to an Olympus C-5060 digital camera (Olympus, Tokyo, Japan) at 10× amplification. Muscle fiber typing using the NADH-TR technique was classified into oxidative and glycolytic, and fibers were identified by the alkaline ATPase technique as slow (type I), intermediate (IIA), and fast (type IIB) (Fig. 1). The total number of each fiber type for each stains, was determined using the Image J program and expressed as percentage of the total number of fibers scored, and the cross-sectional area (CSA, µm2) was measured in sample from the 10th intercostal space of the LT muscle in 200 randomly selected fibers of each fiber type stained with NADH-TR and alkaline ATPase according to described by López-García et al. [17]. Additionally, muscle fiber density was determined by counting the number of each fiber type in 50 000 µm2 of muscle sections according to the method described by Valenzuela-Grijalva et al. [18]. All data were determined and recorded using Image J version 1.45s software (NIH, Bethesda, MD, USA).
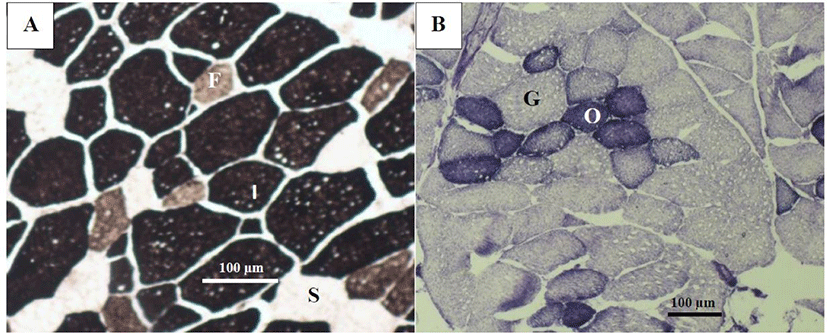
The total RNA of samples from the LT muscle was obtained according to the methodology described by Baxa et al. [19]. A sample of muscle (200 mg) was added into 2 mL tubes (Lysing Matrix A tubes, MP Biomedicals, Solon, OH, USA) with 1 mL of Trizol reagent (Life Technologies, Carlsbad CA, USA) and homogenized for 60 s (FastPrep-24-5G, MP Biomedicals), incubated for 2–3 min at 2°C, and then centrifuged for 10 min at 12,000×g at 4°C. The upper aqueous phase was transferred to a new microtube with 0.3 mL of chloroform, incubated for 3 min at 2°C, and centrifuged for 15 min at 12,000×g at 4°C. The upper aqueous phase was recovered and transferred into a new microtube with 0.3 mL of chloroform and centrifuged for 15 min at 12,000×g at 4°C. Next, the upper aqueous phase was recovered, Trizol (0.5 mL) was added, and the tube was incubated for 5 min at 2°C and later was added 0.3 mL of chloroform and mixed for 15 s. The sample was incubated for 3 min at 4°C and centrifuged for 15 min at 12,000×g at 4°C. The aqueous phase was collected, transferred into a new microtube with isopropanol (0.5 mL), and incubated for 10 min at 4°C. Subsequently, the sample was centrifuged for 15 min at 12,000×g at 4°C, the isopropanol was removed, and the RNA pellet was suspended in 1 mL of 75% ethanol, centrifuged for 5 min at 7,500×g at 4°C. The supernatant was discarded, and the RNA pellet was suspended in 30 µL of diethyl pyrocarbonate (DEPC) water and stored at −20°C for subsequent analysis.
The RNA concentration was determined by measuring the absorbance at 260 and 280 nm using a NanoDrop spectrophotometer (ND-1000 spectrophotometer, NanoDrop, Wilmington, DE, USA). A A260/A280 ratio of ~2.0 was used to assess RNA purity. The integrity of total RNA was evaluated by electrophoresis (65V, 40 min) in a 2% agarose gel (agarose; Sigma-Aldrich). The RNA was treated with a DNAse-I recombinant-RNAase-free kit (Sigma-Aldrich) to remove genomic DNA from the pellet.
The gene expression of β2-AR, MHC-I, MHC-IIX, and IGF-I in muscle tissue were determined using Real-time (RT)- quantitative polymerase chain reaction (qPCR). Hence, ribosomal protein s9 (RPS9) gene was used for normalization [20]. Retro-transcription to cDNA and RT-PCR reaction were performed in one step using 2X One-Step Brillant II QRT-PCR Low Rox Master Mix reagent and reverse transcriptase enzyme (Agilent Technologies, Santa Clara, CA, USA), 1 µL of TaqMan probe and 0.8 µL of RNA template (400 ng of RNA). The lamb-specific β2-AR, MHC-I, MHC-IIX, IGF-I, and RPS9 forward, reverse, and TaqMan detection probes were designed using published GenBank sequences (Table 2). All sequences were synthetized using Single Tube TaqMan Gene Expression assays (Applied Biosystems Integrated DNA Technologies).
Real-time quantitative analysis was performed using the StepOne™ RT-PCR system (Applied Biosystems, Foster City, CA, USA) following the conditions recommended by the manufacturer’s protocol: 1 cycle of 30 min at 50°C, 1 cycle of 10 min at 95°C, 40 cycles of 15 s at 95°C, and 40 cycles of 1 min at 60°C. PCRs were evaluated in triplicate, non-template controls were included, and the results were normalized to RPS9 expression levels. Relative gene expression was calculated using the 2-ΔΔCt method, and data were expressed as relative units [21].
Data on growth performance, carcass characteristics, histoenzymatic parameters, and gene expression were analyzed with a general linear model-analysis of variance for a randomized complete design. The model included treatments as a fixed effect, and the initial body weight of animals as a covariate for growth performance and carcass trait variables. Mean comparisons were performed using the Tukey-Kramer test. Statistical significance was considered at p < 0.05. All data were processed using the statistical package NCSS version 11.
RESULTS
The results of the feedlot performance are presented in Table 3. The final body weight was affected by treatments (p < 0.05), where FA doses were higher than the control, indicating an additive effect of FA. The ADG of animals fed with FA was higher than the control (p < 0.05), and the ADG of animals fed with FA or ZH was similar (p > 0.05). The feed intake was not affected by treatments (p > 0.05), with values ranging between 1.10 and 1.26 kg d-1. Similarly, feed conversion was similar among treatments (p > 0.05).
Temperature-humidity index (THI): THI ≤ 74, Te = 20.68 ± 2.17°C, RH = 42 ± 9.58%, THI = 65.72 ± 2.55 units.
The carcass characteristics of lambs supplemented with FA and ZH are presented in Table 3. The HCW and dressing percentage were not modified by the treatments (p > 0.05). Animals supplemented with ZH had higher LT area than animals in the other treatments (p < 0.05). The carcass fat thickness was not modified by treatments (p > 0.05), with values that ranged between 1.80 and 2.85 mm for all treatments. The pH at 45 min and 24 h postmortem did not change among treatments (p > 0.05), with the pH range at 45 min from 6.38 to 6.48, and at 24 h from 5.59 to 5.71.
Transversal sections of muscle stained with NADH-TR differentiated the type of fibers according to their metabolism (oxidative and glycolytic), and ATPase staining identified fibers as slow or type I, intermediate or type IIA, and fast or type IIB fibers according to their contractile characteristics.
The treatments did not affect the percentages of oxidative and glycolytic fibers (p > 0.05), with percentages between 41.10%–42.64% and 57.35%–58.89%, respectively (Table 4). The CSA of oxidative fibers was larger in the FA and ZH treatments than in the control (p < 0.05); both FA and ZH increased the CSA between 800 and 1,000 µm2 compared to the control. Therefore, CSA in oxidative fibers in the FA treatments was similar to that in the ZH treatment (p > 0.05).
The percentages of fiber types differentiated by the ATPase staining method were similar among treatments (p > 0.05). The percentages of slow fibers ranged between 9.19% and 12.34%, and intermediate and fast fibers fluctuated from 49.28% to 55.62% and from 33.65% to 41.52%, respectively (Table 5). The CSAs of the slow and intermediate fibers were not affected by the treatments (p > 0.05). The CSA of fast fibers was higher in the ZH treatment than in the other treatments (p < 0.05).
The treatments did not affect the fiber density (p > 0.05). However, FA300 and ZH had the lowest density values, which is in accordance with the higher CSA values, although this was not significant (Table 5).
The mRNA abundance of β2-AR, MHC-I, MHC-IIX, and IGF-I are shown in Figs 2, 3, 4, and 5. There was an effect of ZH on the mRNA concentrations of β2-AR (p < 0.05), and ZH treatment increased the β2-AR expression three-fold for the FA doses and control groups (p < 0.05). The mRNA concentrations for β2-AR in the FA300, FA600, and control groups were similar (p > 0.05).
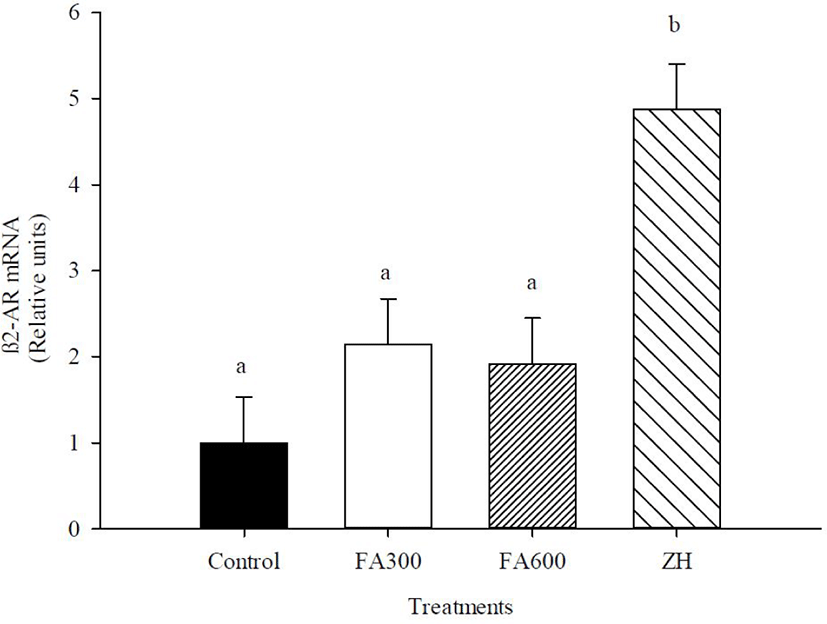
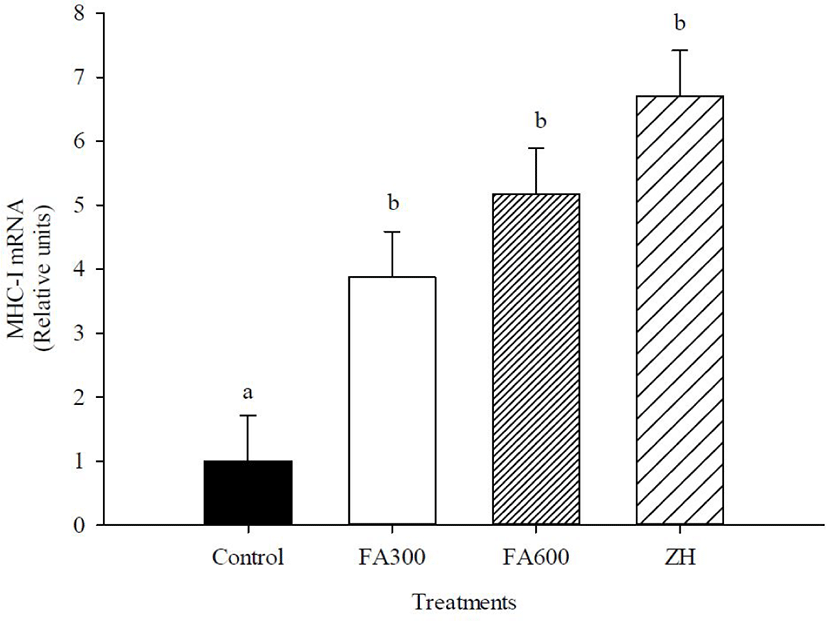
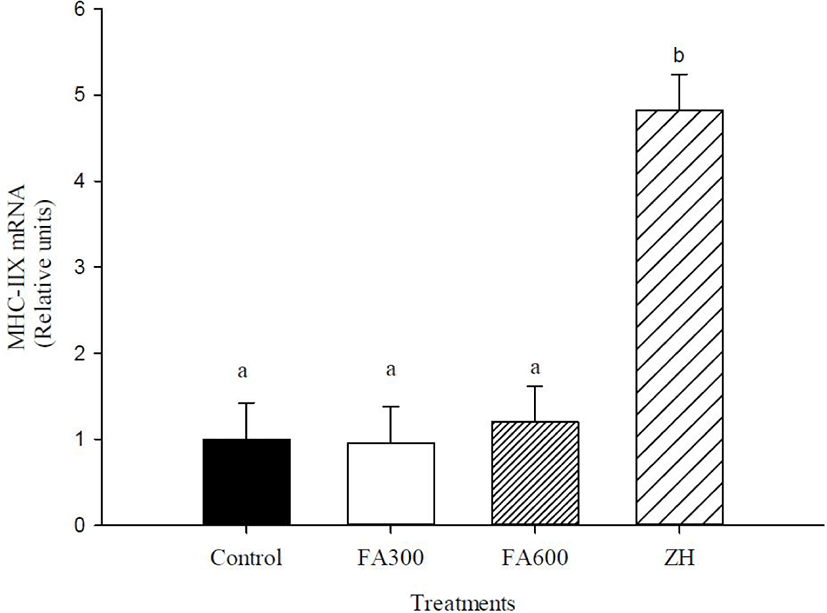
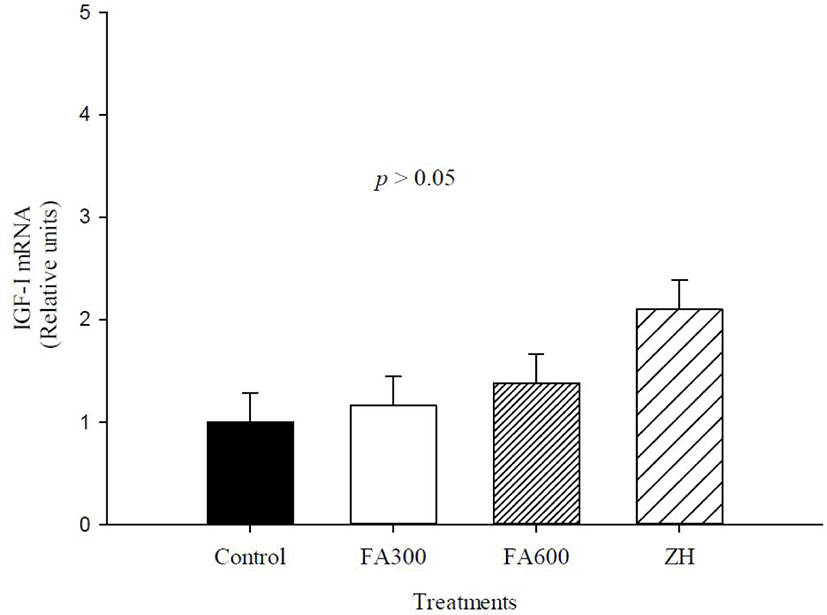
The FA300, FA600, and ZH treatments increased mRNA abundance of MHC-I compared to the non-supplemented animals (p < 0.05), with the mRNA values of experimental treatments fluctuating between 3 and 6.7 relative units. MHC-IIX gene expression changed only in the ZH treatment, and the synthetic molecule increased the mRNA abundance up to 3.7 relative units in the FA300, FA600, and control groups (p < 0.05). The mRNA levels of IGF-I were not altered by the experimental treatments, and the values oscillated between 1 and 2.04 relative units (p > 0.05).
DISCUSSION
The feedlot performance of the FA and ZH treatments in the present study was similar with other studies that used ZH in finishing lambs, with increase in ADG and improved feed conversion [22,23]. These modifications on growth performance are well documented as a repartitioning effect caused by β-AA and are the most important production parameters [24]. However, other studies in lambs have reported contrary results. Dávila-Ramírez et al. [25] and Macías-Cruz et al. [26] evaluated feedlot performance in hair lambs with 10 mg d−1 of ZH and did not observe changes in ADG and feed efficiency, due to environmental factors as heat stress, that reduce voluntary feed and metabolizable energy intake [27].
Studies related to the effects of FA supplementation on productive species are limited and inconsistent. Results of the present study agree with a previous study of finishing heifers supplemented with FA (2,250 or 4,500 mg FA d−1) where an increase of ADG and feed conversion was observed compared to non-supplemented animals [6]. Another study on ewe lambs reported an increase on wholesale cut yields when they were supplemented for 40 days with 250 mg kg−1 of FA under extreme heat stress [7]. In contrast, a previous study observed no effects on feedlot performance when ewe lambs were supplemented with 300 mg d−1 FA for 34 d under heat stress conditions [28].
The mode of action of FA has not yet been completely elucidated and a study in lactating cows supplemented with FA associated a hormonal mechanism as result of an increase of serum somatotropin [29]. By other hand, in a study on finishing pigs supplemented with FA, hypothesized a β-AA mechanism, due to improvements in final body weight, feed conversion, carcass yields and overexpression of β-AR, IGF-I, and MHC-I, similar to pigs with ractopamine hydrochloride treatment [30], however, is necessary more studies focused on myogenic regulatory factors (MRFs) and endogenous proteolytic systems to confirm an anabolic mechanism of FA. In addition, a modulating effect of the rumen microbiota has been suggested as other mode of action of FA because there is evidence that isolated extracts from plants directly affect the rumen or intestinal microbial ecosystem, generating beneficial changes in volatile fatty acids, which are the first energy source in ruminants [1,31,32].
Concerning the carcass parameters, the pH parameters were similar for all treatments and within normal values for the fresh meat of lambs [33]. Only the ZH treatment showed a significant increase in LT muscle area, which has been well documented in other reports and explained by protein synthesis activation and repartitioning effect in LT muscle [8,34].
Despite the important benefits of FA in the feedlot, the carcasses of these animals were similar to those of the control group. Similar results were reported by Macías-Cruz et al. [35] except in the LT area; therefore, ZH supplementation in hair lambs does not result in changes in carcass variables. In contrast, in pigs supplemented with FA, fat thickness was significantly reduced by supplementation with 50 mg FA kg−1 of feed, suggesting a lipolytic effect [5]. An explanation of the contrasting results in the feedlot and carcass with FA in the present study could be that compensatory growth from the high-energy diet, the age of the lambs (4 months), or the different origin of the animals caused these changes [36]. In the present study, it was not possible to measure the weight of organs and non-carcass components. The literature indicates that β-AA has little effect on organs and non-carcass components such as the liver, intestine, and skin, or the redirection of nutrients from the organs for muscle development; in this sense, is necessary further studies because FA metabolism could be distinct in gastrointestinal tract in ruminants and there is also the possibility that orally ingested FA can be degraded or transformed in the rumen, resulting in a diminished FA effect in the skeletal muscle [37].
Therefore, precise experiments should be performed on the kinetics of ruminal microbiota, volatile fatty acid proportions, metabolic routes, and pharmacodynamics to establish the action mechanism and effective FA doses.
Similar to the present study, FA caused a hypertrophy effect in pigs supplemented with 50 mg d−1, where the CSA increased fast twitch fibers owing to the upregulation of MHC-IIX and IGF-1 genes [30]. Furthermore, in male rats (12 weeks old) supplemented with 0.5% apple polyphenols for 8 weeks, an increased CSA in oxidative fibers was observed due to the upregulation of MHC type I [38]. A recent study in 21-day-old piglets demonstrated that FA could promote muscle fiber type conversion from fast-twitch to slow-twitch via the Sirt1/AMPK/PGC-1α signaling pathway, moreover a decrease of lactate dehydrogenase (LDH) activity, that is positively associated with glycolytic fibers confirming that FA could promote the transformation of muscle fiber type from fast-twitch to slow-twitch. [39].
β-AA is used during the finishing phases of productive species and these compounds generate increases in muscle mass and carcass yields caused by muscle hypertrophy [8]. The treatments in the present study presented similar behavior as those lambs supplemented with β-AA cimaterol, which caused an increase in CSA by 50% compared to the non-supplemented group and there were no changes in the proportion of oxidative and glycolytic fibers [40]. The same effect was presented in steers with ZH + anabolic implants that contained CSA of fibers type I and IIX in longissimus lumborum that were higher than that of the non-supplemented group [41]. One explanation for this can be attributed to β2-AR activation of the stem cells generating overexpression of MHC protein isoforms and greater muscle development [8]. The present study results did not show differences between fiber ratios; however, it can note that FA and ZH treatments have ratios of glycolytic slightly above, that could suggest a light muscle fiber transition as occurs with β-AA molecules [11,41], and repartitioning the energy from nutrients to glycolytic fibers, which could be reflected in more wholesale cuts yields [8].
To the best of our knowledge, this is the first study to evaluate the effect of FA inclusion on gene expression in hair lambs. Previous studies tested FA in bovine satellite cells (in vitro) and the muscle of pigs (in vivo) and found high levels of mRNA relative abundance for β2-AR, similar to β-AA [30,42]. These differences in our study might be because FA does not bind to β2-AR and there might be other receptors or other mechanisms that recognize FA and promote muscle deposition. A recent study indicates that FA has mitochondrial and enzymatic pathways that promote fiber type conversion from fast-twitch to slow-twitch and myogenic regulators targeting hypertrophy in oxidative fibers [39]. Therefore, further research is required to confirm the initial action mechanism of FA to promote growth in lambs.
β-AAs are recognized by membrane receptors in skeletal muscle (β1-AR and β2-AR) and increase cell transcription and translation directed to muscle protein synthesis, lipolysis, and the rate reduction of protein degradation [8]. The literature indicates that the β2-AR subtype showed an increase in mRNA levels more than β1-AR in steers after ZH treatment, which confirms our results with ZH in hair lambs [43,44].
The results of mRNA abundance of MHC-I with FA and ZH treatments are in accordance with the increase of CSA for slow or oxidative fibers. In other studies whit FA extracted from gamma oryzanol, was increased muscular straightness and induced larger increases in exercise capacity and antifatigue, which were related to the characteristics and properties of slow fibers and oxidative metabolism, including fatigue resistance [45,46]. Similarly, the capillarity of muscle fibers directly influences the morphology, development, and maintenance of muscle fibers. Capillaries surround the muscle fiber, and the number of capillaries is higher in type I fibers (oxidative) than in type II fibers (glycolytic); therefore, the supply of nutrients and energy can be directed to anabolism [47,48] and this mechanism helps increase MHC-I levels with FA. MHC-IIX expression levels were not modified in hair lambs with FA300 or FA600 treatments, coinciding with the null differences found in the CSA of fast and glycolytic fibers. Therefore, the growth promoter of FA is limited only by MHC-I expression, which is supported by studies showing that FA improved muscle strength in steers and increased the relative abundance of mRNA for MHC in satellite cells [25,49]. Other studies have evaluated the gene expression of antioxidant enzymes such as glutathione peroxidase and catalase or immune system as pro-inflammatory cytokines and showed that FA helped improve the health status of animals, which could be another mode of action for growth promotion [50,51,52].
On the other hand, the mode of action of FA, also could be hypothesized like a mimic of β-AA, since recently a study with the phytochemical higenamine from herbal extracts evidenced an activation of β1-AR, β2-AR and G proteins in cultured myocardial cells [53]. However, more studies are required to confirm this possible mechanism of action.
Respect to effect of ZH, a study with cimaterol hydrochloride in hair lambs showed muscle hypertrophy in slow fibers, in comparison the fiber size increased the MHC-I overexpression compared to the non-supplemented group [54]. The relative abundance of MHC-IIX in the ZH treatment in the present study was consistent with other studies in bovines with ZH treatment, which altered the levels of MHC-IIX in LT and Semimembranosus muscles [19,55]. Our results with ZH corresponded with the usual mode of action of β-AA in bovines, provoking a transition from oxidative fibers to predominantly glycolytic fibers, which was influenced by an alteration in the MHC-IIA and MHC-IIX isoforms with a major effect or improvements on feed efficiency, ADG, carcass dressing, and primary cuts [24]. This effect is consistent with the increase in the glycolytic fiber size with ZH, confirming the action mechanism of β-AA compounds reported in ruminants, which promotes muscle hypertrophy.
The growth promoter action for IGF-I activation is via the proliferation of satellite cells and muscle mass growth, and studies of mRNA abundance of this gene have shown such an effect [56,57]. Our results with FA did not present significant values and contrast with a study on pigs supplemented with FA, where mRNA abundance was higher than the β-AA treatment [30].
In ruminants, several studies have evaluated this hormonal indicator, without changes in IGF-I with β-AA treatment. Satellite cells of bovines were probed with ZH, and the relative abundance of IGF-I mRNA at 192 h was zero [42]. In addition, ewe lambs supplemented with clenbuterol hydrochloride had incremented muscle weight but not plasma IGF-I concentration [58]. Dikeman et al. [59] indicated that the administration of β-AA reduced IGF-I mRNA in skeletal muscle and circulating IGF-I because IGF-I is a stimulator of satellite cell proliferation with no apparent change in DNA content and limits the capacity to sustain muscle hypertrophy.
CONCLUSION
FA administration in hair lambs produced a muscle hypertrophy effect on productive and physiological variables; however, this was not reflected in the carcass characteristics. Future studies should be carried out to confirm whether an activation of β-AR occurs and to be able to associate it with the increase in muscle deposition after supplementation with FA in hair lambs, or to investigate intracellular pathways involved in this response. For ZH, the action mode as a growth promoter in lambs was confirmed, which was recognized in β2-AR and caused an upregulation of MHC isoforms and an increase in the size of both fiber types. In conclusion, FA can be one of the candidate agents to improve the muscle growth in intensive feedlot lambs; however, further studies are required to elucidate the effective doses that increase the economic benefit of wholesale cut yields.