INTRODUCTION
The rise in global temperature in recent decades has negatively affected agriculture and food supply. More than half of dairy cows live in subtropical and tropical areas that have a temperature-humidity index (THI) which tends to reach 68 or more, and the risk of heat stress in dairy cows is inevitable [1]. Heat stress leads to a 10%–35% decline in milk yield [2] and an estimated 5.4% loss in the monthly income of dairy farmers during summer [3]. Therefore, understanding the mechanism by which heat stress induces a decrease in milk protein synthesis is crucial to improve the milk production potential of dairy cattle during summer.
Heat stress, which reduces the dry matter intake (DMI) of dairy cows, has been traditionally considered as the major cause for the decreased milk production potential under hyperthermic environments [4,5]. However, for the past few years, the experiment of pair-fed to non-heat-stress cows confirmed that the decrease in milk yield and milk composition was only partly caused by the reduction of DMI [6,7]. Further studies indicated that the decrease in the production of milk protein induced by heat stress was specifically caused by a decline in the activity of mammary protein synthesis rather than a decrease in milk yield [6,8]. Heat stress promotes the consumption of extra-mammary amino acids, including urinary nitrogen excretion and rumen microbial protein synthesis, in dairy cows, which reduces the amount of amino acids available to the mammary gland for the synthesis of milk proteins [9]. Transcriptome analysis indicated that heat stress strongly inhibited the amino acids metabolic activity in the mammary tissue, and the data suggested that the decreased availability of amino acids resulted in a decreased synthesis of milk proteins [10]. In addition, hyperthermia reduced cell viability in bovine mammary epithelial cells (BMECs) [11] and alveoli number in the lactating mammary gland [12]. Hyperthermia negatively regulates the number and activity of mammary gland cells, thereby contributing to a decrease in milk production under high-temperature stress [13]. However, to our knowledge, the influence of heat stress on the transport of amino acids in the mammary gland of lactating cows is insufficient in the existing literature.
As the precursor of the synthesis of milk proteins, amino acids perform critical functions in the regulation of physiological functions [14]. For instance, the branched-chain amino acids, such as isoleucine, valine, and leucine regulate nutrition metabolism, immunity, and energy homeostasis in mammals [15]. Methionine (Met) and arginine (Arg) may stimulate the mammalian target of rapamycin complex 1 (mTORC1) and promote protein synthesis [16]. Dietary supplementation of Met could increase the milk protein concentration and improve milk production in dairy cows [17,18]. Under hyperthermic conditions, enhanced supply of Met and Arg had a positive effect on milk protein synthesis in heat-stressed BMECs [19], and supplementation of Met helped maintain milk composition in heat-stressed lactating Holstein cows [20]. We hypothesized that the heat stress-induced reduction in milk protein synthesis was due to the decrease in the uptake of amino acids by mammary cells. Heat stress refers to a sequence of non-specific physiological responses to maintain a constant body temperature [4]. In vitro, apoptosis induced by hyperthermia is also considered a response to heat stress [21,22]. Bovine mammary epithelial (MAC-T) cells are well-known mammary epithelial cell line and retain the phenotypic characteristics of BMECs [23,24], have been used extensively to study apoptosis in the immune response or oxidative stress [25,26], milk protein synthesis, and mammalian lactation [27,28]. Thus, we primarily aimed to examine the impact of heat stress on the synthesis of milk protein by incubating MAC-T cells at a hyperthermic temperature (42°C).
MATERIALS AND METHODS
Frozen bovine MAC-T cells were recovered and allowed to grow in 75 cm2 cell culture flasks at a temperature of 37°C and 5% CO2 concentration to obtain enough biological material for subsequent analysis. Cells at 80%–90% confluency were transferred into 6-well plates (1.2~1.5×105 cells per well, Thermo Scientific, Waltham, MA, USA). To culture MAC-T cells, we utilized the complete medium consist of Dulbecco’s modified Eagle’s medium (DMEM,Thermo Scientific) accompanied by 10 percent fetal bovine serum (FBS; Thermo Scientific), 100 μg/mL streptomycin, and 100 IU/mL penicillin G (Sigma Aldrich, St Louis, MO, USA). After every 48 hours, the culture medium was replaced. The cells were washed using phosphate-buffered saline (PBS, Thermo Scientific) three times and the medium was changed until the confluency was 80% to 90%. Then, MAC-T cells were divided into two groups (n=6 replicas for each treatment) and subjected to incubation at 37°C (CON) or 42°C (heat stress [HS]) for 6 h, respectively. The incubation temperature and time were set at 42°C and 6 h, based on a previous study by Collier et al. [29] where the mRNA concentration of heat shock protein 70 (HSP70) was considerably elevated in BMECs within 1 and 2 h, and it attained a peak after 4 hours following the exposure of the cells to 42°C.
The viability of the cells was assessed utilizing an MTT test kit in accordance with the instructions stipulated by the manufacturer. Briefly, 100 μL medium containing MAC-T cells (2×104/mL) were transferred into 96-well culture plates, followed by treatment at 37°C or 42°C for 6 hours. Afterward, they were subjected to incubation for 16 hours at 37°C after being incubated for 4 hours with 10 μL of MTT staining solution within every well plate. Subsequently, 100 μL of the formazan crystals were added in all the wells at 37°C for 4 h until completely dissolved, and a microplate reader (Bio-Rad, Hercules, CA, USA) was utilized to determine the optical density (OD) at 570 nm. Cell apoptosis rate was determined utilizing an Annexin V-FITC/PI apoptosis detection kit (4A Biotech, Beijing, China) in compliance with the protocols provided by the manufacturer. The excitation wavelength was 525 nm (Annexin V-FITC, green fluorescence), and the emission wavelength was (595 nm PI, red fluorescence). The results were evaluated utilizing the Cell-Quest software (BD Biosciences, Franklin Lakes, NJ, USA).
The Steady Pure Universal RNA Extraction Kit (Accurate Bio, Hunan, China) was utilized to extract and purify total RNA from MAC-T cells according to the instructions provided by the manufacturer. The NanoDrop 2000 spectrophotometer (Thermo Scientific) was utilized to determine the purity as well as the concentration of the isolated RNA. Additionally, the integrity of the RNA was examined utilizing an Agilent 2100 Bioanalyzer (Agilent Technologies, Santa Clara, CA, USA). The samples that had an RNA Integrity Number (RIN) ≥ 7.0 underwent dilution to 100 ng/μL with RNase-free water. The reverse transcription of the diluted RNA samples to cDNA was performed utilizing the Prime-Script™ RT-PCR reagent Kit with gDNA Eraser (Takara, Tokyo, Japan) in accordance with the protocols stipulated by the manufacturer. For additional analysis, RNase-free water was utilized to dilute the cDNA at a ratio of 1: 5.
With the aid of SYBR Premix Ex Taq reagents (TaKaRa, Dalian, China), we conducted qRT-PCR in an ABI 7500 real-time thermocycler (Applied Biosystems, Foster City, CA, USA), as earlier described [30,31]. To normalize the target gene expression, the reference gene utilized was glyceraldehyde-3-phosphate dehydrogenase (GAPDH) [31]. Table 1 contains a list of sequences of all primers, which were commercially manufactured by Invitrogen (Shanghai, China). The relative mRNA target genes expression was computed utilizing the comparative cycle threshold (2−ΔΔCt) method [32]. Each of the biological samples was replicated three times on a 96-well real-time PCR plate (Applied Biosystems).
GAPDH, glyceraldehyde-3-phosphate dehydrogenase; BCL-2, B cell leukemia/lymphoma 2; BAX, Bcl-2-associated X protein; Caspase-3, cysteinyl aspartate specific proteinase-3; Caspase-9, cysteinyl aspartate specific proteinase-9; HSP70, heat shock protein 70; HSP90B1, heat shock protein 90B1; CSN1S1, casein alpha s1; CSN2, casein beta; CSN3, casein kappa; SLC7A5, solute carrier family 7, member 5; AKT1, serine-threonine protein kinas 1; mTOR, mammalian target of rapamycin; RPS6, ribosomal protein S6; RPS6KB1, ribosomal protein S6 kinase B1; SLC38A3, solute carrier family 38, member 3; SLC38A9, solute carrier family 38, member 9; SLC38A2, solute carrier family 38, member 2.
The Western blot analysis was conducted in the same way as previously reported [33]. Briefly, MAC-T cells were solubilized in radioimmunoprecipitation assay (RIPA) Lysis and Extraction Buffer (Invitrogen, Waltham, MA, USA) to obtain total protein. After denaturation at high temperature, the protein samples extracted from cells were isolated utilizing sodium dodecyl sulfate-polyacrylamide gel electrophoresis (SDS-PAGE) and subsequently loaded onto a nitrocellulose membrane. Blocking of the membrane was carried out using 5% skimmed milk generated in Tris-buffer, followed by incubation using primary antibodies (Complete details are listed in Table 2) over the night at 4°C. Subsequently, incubation of the membrane was conducted using horseradish peroxidase (HRP)-conjugated anti-rabbit IgG secondary antibody (Complete details are listed in Table 2) at ambient temperature for 4 hours. Finally, detection of the blot was done utilizing ECL™ Western Blotting Detection Reagent (GE Healthcare, Piscataway, NJ, USA) and visualization of the proteins was achieved using enhanced chemiluminescence (Amersham Biosciences, Piscataway, NJ, USA). The intensity of β-actin was utilized as an endogenous control.
RESULTS
As illustrated in Fig. 1, MAC-T cells thermally treated at 42°C for 6 h showed a 28.81% decrease in cell viability as opposed to the CON group (p < 0.05). Furthermore, heat stress considerably elevated the early apoptotic rate (14.72% vs. 3.18%) and late apoptotic rate (3.01% vs. 0.91%) of MAC-T cells (p < 0.05).
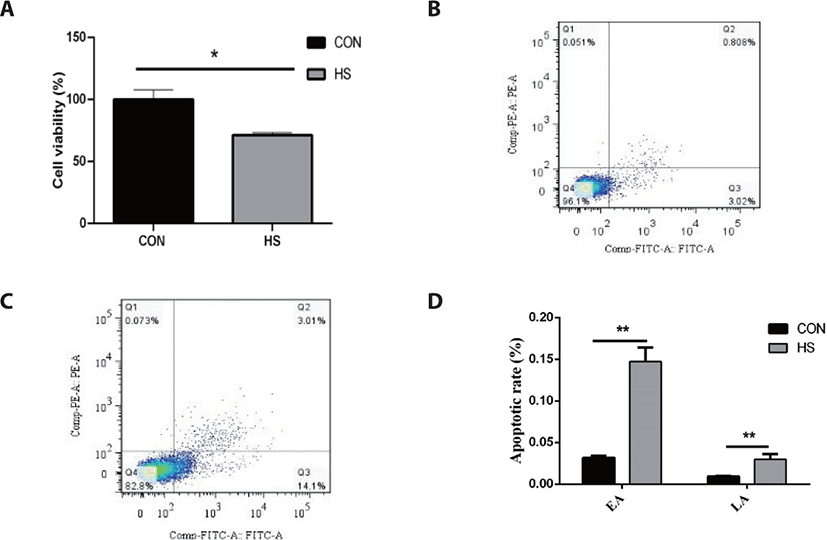
Heat stress greatly elevated the gene expression of HSP90B) and HSP70 (p < 0.01) in MAC-T cells (p < 0.01; Fig. 2). Bcl-2-associated X protein (BAX) gene expression was greatly elevated in response to heat stress (p < 0.01), while the B-cell lymphoma 2 (BCL2) gene expression was not affected. In addition, heat stress considerably elevated the caspase-9 and caspase-3 gene expressions (both p < 0.05).
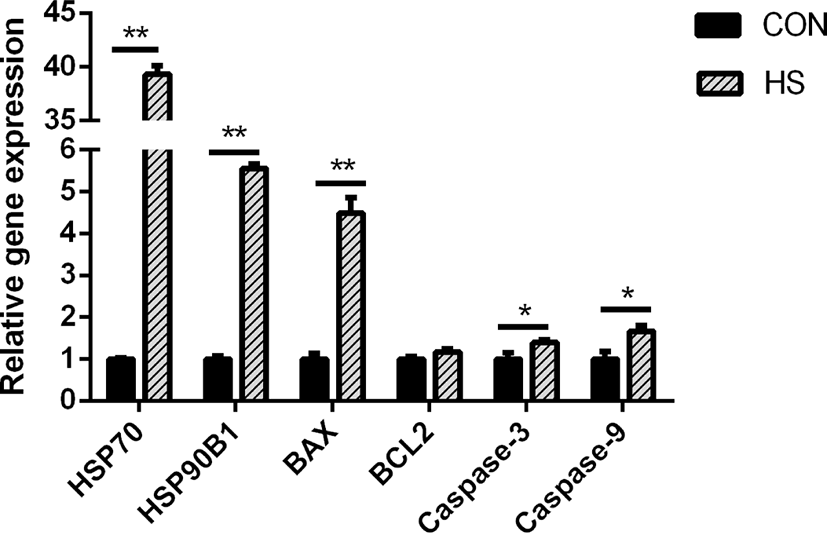
The HSP70 protein expression was substantially elevated in MAC-T cells upon exposure to heat stress (p < 0.01; Fig. 3). Heat stress substantially elevated the BAX protein expression (p < 0.01) while decreasing that of BCL2 (p < 0.05).
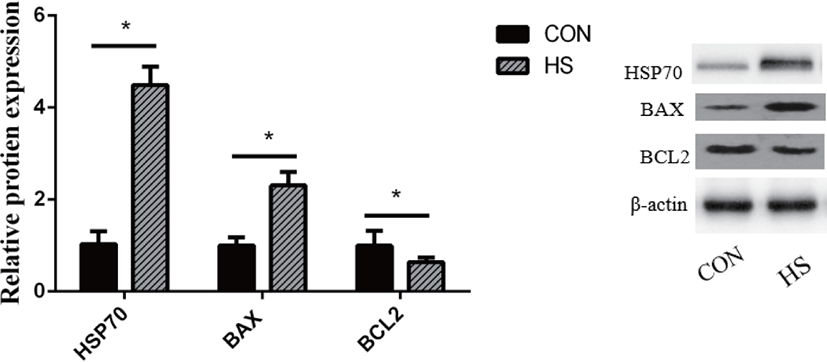
Heat stress considerably reduced the gene expression of ribosomal protein S6 (RPS6, p < 0.05), AKT serine/threonine kinase 1 (AKT1, p < 0.05), and ribosomal protein S6 kinase B1 (RPS6KB1, p < 0.05) (Fig. 4).
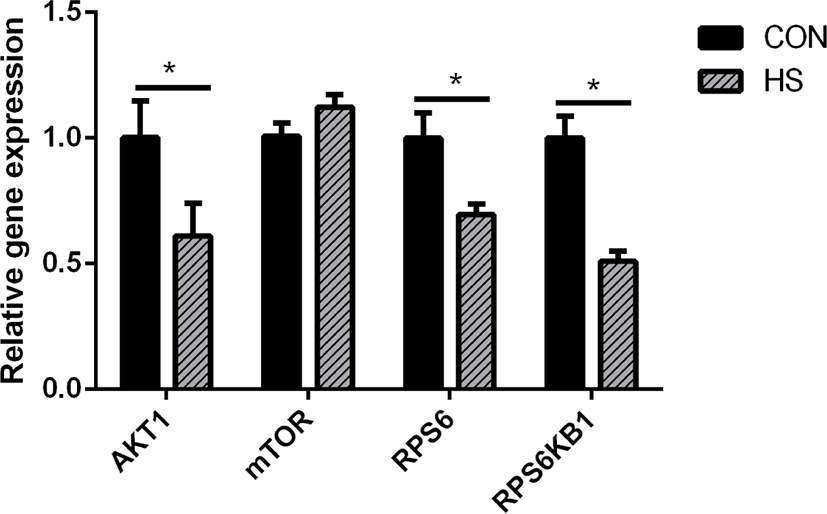
Heat stress significantly downregulated the gene expression of casein kappa (CSN3, p < 0.01) and casein alpha s1 (CSN1S1, p < 0.05), casein beta (CSN2, p < 0.05). Moreover, heat stress downregulated the gene expression of solute carrier family 38 member 2 (SLC38A2, p < 0.05), SLC38A9 (p <0.05), SLC38A3 (p < 0.05), and SLC7A5 ( p < 0.05) as shown in Fig. 5.
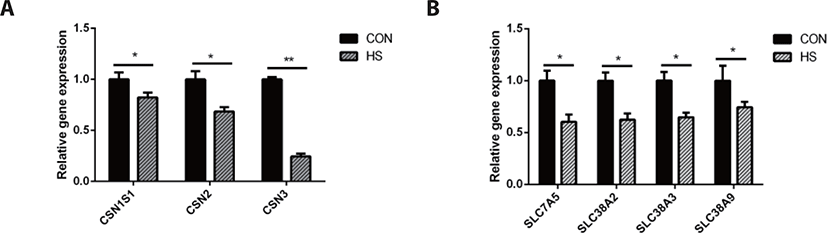
DISCUSSION
High temperature can induce DNA damage, mitochondrial dysfunction, and abnormal gene expression and protein synthesis, eventually leading to cell death [34-36]. Liu et al. [37] showed that heat-stressed BMECs were characterized by the presence of condensed nuclei and cytoplasmic vacuoles. Moreover, they found that cells released a large number of cellular fragments into the medium and exhibited cytolysis and disorganization [37]. Hyperpyrexia could cause a decrease in the total number and activity of BMECs by inducing apoptosis [38]. During heat stress, cells mount a series of regulatory stress responses to maintain cell homeostasis [39]. For instance, as an adaptive cellular response to heat stress, cells rapidly upregulate the transcription and translation of HSPs to protect against protein aggregation and degradation [40], thereby restoring the normal function of the mammary gland. Both HSP90 and HSP70 perform mostly anti-apoptotic functions [41]. However, heat stress also induces the expression of pro-and anti-apoptotic members of the Bcl-2 protein family, which are known to regulate cell death [42]. Through the interaction of these proteins, the binding of cytochrome c released from mitochondria to cytosolic Apaf-1 results in the formation of a caspase-activating complex known as apoptosome [42]. The dimerization of caspase-9 within the apoptosome complex activates caspase-3, which results in apoptotic body formation and cellular inactivation through the cleavage specific proteins [43]. During this process, Bak and Bax, the pro-apoptotic Bcl-2 family members, perform a function of positively modulating the cytochrome c release from mitochondria [44], while the antiapoptotic Bcl-2 family members, Bcl-2 and Bcl-xL, suppress its release [45]. In this study, we found that hyperthermia decreased the viability and increased the apoptotic rate of MAC-T cells. The protein and gene expression of BAX was upregulated in the HS group, which is considered a crucial step in the mitochondrial apoptotic pathway [46]. Moreover, the higher expression of HSP70, HSP90B1, caspase-9 and caspase-3 genes and HSP70 protein was observed in the HS group. These results suggested that MAC-T cells underwent apoptosis after incubation at 42°C for 6 h, which might have resulted in a decrease in milk protein synthesis.
As the MAC-T cell line is incapable of secreting milk components, milk protein content could not be detected directly in this study. The CSN2 gene expression is positively associated with milk yield [47]. Hence, the expression of casein genes may be used to evaluate milk yield as an alternative to the evaluation of casein protein synthesis in MAC-T cells [48]. We compared the CSN1S1, CSN2, and CSN3 genes expression, which are the most highly expressed casein genes in milk protein [49], between the HS and CON groups and found that their expressions were significantly decreased in the HS group. These findings corroborate an earlier research report on the mammary gland tissue of heat-stressed lactating dairy cows [50] and another study on heat-stressed BMECs [51]. Therefore, heat stress could directly inhibit the synthesis of casein proteins, and the decrease in the DMI may be partly responsible for the decrease in the synthesis of milk protein in lactating cows under heat stress. Heat stress destroyed the cytoskeleton of BMECs, inhibited the cell cycle [52], and substantially reduced the mTOR signaling pathway activity [53], which is known as the regulator of protein synthesis. As a key upstream modulator of the mTOR signaling pathway, AKT performs a vital function in the maintenance of cell survival and depletion before the induction of apoptosis in fibroblast cells exposed to heat stress for a long term [54]. Hyperthermia decreased the phosphorylation state of AKT, RPS6K1, and RPS6, which are regarded as the upstream and downstream protein factors of the mTOR signaling pathway in MAC-T cells [54]. The suppression of the mTOR signaling pathway may be attributed the reduction of milk protein synthesis.
Amino acids are nutrients essential for the survival of all cell types. They not only serve as the precursor molecules for protein synthesis but can also regulate cellular function. For example, leucine (Leu), glutamine (Gln), and Arg function as signaling factors in the mTOR signaling pathway; serine (Ser), Glu, glycine (Gly), and aspartate (Asp) are necessary for nucleotide synthesis [55,56]. Thus, the normal function of mammary cells depends on the intracellular amino acid supply modulated by amino acid transporters. Interestingly, we also found that the gene expression of amino acid transporters was downregulated by hyperthermia. Amino acid transporters are membrane transporters and the majority of them belong to the solute carrier family of membrane transport proteins. SLC7A5 is a systemic L-type amino acid transporter (LAT1) that exclusively transports essential amino acids [57]. In many cells, the SLC7A5-mediated import of amino acids is essential to maintain mTOR activity [58]. One of the main functions of mTOR is to speed up the translation of mRNA, where amino acids are required as precursors [58]. Thus, the hyperthermia-induced decrease in SLC7A5 gene expression could have caused the decrease in amino acid transport, which inhibited the mTOR signaling pathway activity, eventually resulting in the reduction of lactoprotein synthesis in heat-stressed MAC-T cells. The inhibition of the mTOR signaling pathway significantly reduced the expression of β-casein and LAT1 (encoded by SLC7A5) [59]. The transporters classified as SLC38 family are known as sodium neutral amino acid transporters, which can perform the net transport of neutral amino acids [60]. This family of proteins contributes to maintaining the homeostatic pool of extracellular and intracellular amino acids [61]. These results suggest that the mTOR signaling pathway and amino acid transporters regulate each other to regulate the synthesis of milk protein in mammary cells of dairy cows. In contrast, a reduction in the supply of amino acids may also result in a decline in milk protein synthesis due to the shortage of essential substrates. In this research, the decreased expression of amino acid transporter genes in heat-stressed MAC-T cells might be linked to the decreased synthesis of milk proteins.
CONCLUSION
Hyperthermia induced apoptosis and lowered the expression of mTOR signaling pathway-related genes in MAC-T cells. Additionally, hyperthermia downregulated the expression of amino acid transporter genes, which might decrease the supply of amino acids available to MAC-T cells. Subsequently, the deficiency of amino acids was the root cause for the decreased rate of protein synthesis in MAC-T cells under heat stress. The results from this research may offer novel directions for the development of strategies to alleviate the loss of milk production induced by heat stress.