INTRODUCTION
Since 1950, the poultry industry has been routinely used antibiotics as feed additives at subtherapeutic concentrations to improve growth performance and feed efficiency as well as to maintain health in poultry flocks [1]. However, with the increasing scientific evidence indicating the associations between emergence of antibiotic resistance and antibiotic growth promoters (AGP), several countries acted upon to limit the use of these compounds. Sweden was the first country to ban antibiotics as growth promoters and subsequently the European Union completely prohibited the use of AGP in animal feed since January 1, 2006 [2]. In 2011, Korea, the first Asian country, strictly enforced ban on the use of all AGP in animal feed [3]. India has introduced a drug withdrawal period for livestock production in 2013 (7 days for eggs and 28 days for poultry meat) [4]. In 2015, the state of California passed a bill (Senate Bill 27) enforcing a strict ban on using medically important antimicrobials in animal feeds for both growth promotion and disease prevention [5]. Recently, China [6] and Brazil [7] banned the use of colistin. Similarly, Bangladesh, Bhutan, Indonesia, Myanmar, Nepal, Sri Lanka, Vietnam, and Thailand have also announced national action plans for AGP restrictions [8]. However, these antibiotic restrictions in poultry led to sub-optimal performance and high production costs due to increased morbidity as well as mortality [9]. Moreover, the ban of AGP in poultry feed have markedly increased the use of therapeutic antibiotics due to frequent incidence of diseases in poultry leading to the disruption in normal gut microbiota compositions and functions. Such disruption is called ‘gut dysbiosis’ that impairs host homeostasis [10-12]. Therefore, the poultry industry has been continuously exploring a wide range of new antibiotic alternatives to improve the productivity of birds without compromising their health.
Polyphosphate is a polymer of 10 to 100 orthophosphates (Pi) linked by high-energy phosphate bonds. It exists in various organisms ranging from bacteria to mammals [13]. Polyphosphate plays a role in energy metabolism, survival, regulation of gene expression, translation fidelity, motility, and virulence [14]. It has high buffering capacity, polyanion and sequestering properties, and water-retaining ability [15]. Recently, it has been reported that polyphosphate in bacterial cells can also improve biofilm formationand regulate virulence as well as responses to nutritional and environmental stresses [13,16]. Polyphosphate also plays an important role as a biological active substance in mammals for tissue and bone generation as well as for blood coagulation [14,17]. It has high antimicrobial effects against various Gram-positive bacteria such as Staphylococcus aureus, Listeria monocytogenes, Sarcina lutea, Bacillus cereus, and Lactobacillus, and against fungi, such as Aspergillus flavus [18,19]. This is related to its ability to chelate divalent cations, resulting in cell division inhibition and loss of cell wall integrity [18]. Polyphosphates may also inhibit microorganisms by forming potentially inhibitory compounds (e.g., superoxide) or disrupting intracellular metabolism, adenosine triphosphate (ATP) generation, or protein folding [20]. Due to antimicrobial activity,polyphosphate has long been used in food industry as a decontaminating agent, especially in animal products [15]. However, most of the studies on polyphosphate have been performed in vitro using specific pathogenic microbes and eukaryotic cells [19,21,22].
One of the major challenges posed by the non-antibiotic broiler production is the greater susceptibility to bacterial diseases. Some of the common bacterial poultry pathogens include Escherichia coli, Enterococcusspp., S. aureus, Salmonellaenterica,and Campylobacter spp. [23]. Studies on long chain polyphosphate in animals, especially livestock, are very limited. Therefore, the objective of this study was to investigate the antimicrobial and anti-biofilm activities of sodium long chain polyphosphate (SLCPP) against poultry pathogenic bacteria in vitro and the dietary supplemental effect of SLCPP on productivity, organ characteristics, intestinal microflora, and blood metabolites of broilers. Results of this study will provide important information regarding whether SLCPP could be used as an alternative of AGP.
MATERIALS AND METHODS
Sodium polyphosphate (Nan+2PnO3n+1) with an average chain length of 130 Piwas provided by RegeneTiss (Kunitachi, Japan) and used for in vitro and in vivo experiments.
A total of seven pathogenic bacterial strains were used for determining antimicrobial activities of SLCPP. E. coli O157:H7 ATCC 35150, Listeria monocytogenesKCCM 40307, Salmonella entericaser.Gallinarum ATCC 9184, S. entericaser.Pullorum SP4, Pseudomonas aeruginosaPA01, and Shigella sonnei KCTC 2518 were provided by Korea Research Institute of Bioscience and Biotechnology (Cheongju, Korea). Klebsiella pneumoniaewas provided by Gyeonggi-do Environmental Research Institute (Suwon, Korea). E. coli O157:H7, S. entericaser.Gallinarum, S. entericaser.Pullorum SP4, K. pneumoniae,and S. sonnei were grown in Luria-Bertani (LB; Difco, Franklin Lakes, NJ, USA) broth. L. monocytogenes and P. aeruginosa were grown in brain heart infusion (BHI; Difco) broth and nutrient broth (Difco), respectively. Cultures were grown aerobically at 37°C for 16 h under shaking conditions (100 rpm).
SLCPP was dissolved in sterile distilled water to achieve final concentrations of 10 mg/mL, 50 mg/mL, and 100 mg/mL. It was then adjusted to pH 7 and filter sterilized. All the freshly prepared bacterial cultures (~1×108–9 CFU/mL) were swabbed onto respective agar plates using sterilized cotton swabs. Aliquot (100 µL) of SLCPP at three different concentrations was then placed into wells (6 mm diameter) of agar plates. Plates were then incubated at 37°C for 16 h to observe the zone of inhibition.
The potential of SLCPP to prevent initial cell attachment was determined through measuring the percentage of biofilm inhibition as described by Jadhav et al. [24] and Lee et al. [25] with some modifications. Five pathogenic bacterial strains were selected for anti-biofilm activity evaluation based on their results of agar well diffusion assay. Test solutions of SLCPP were prepared in LB, BHI, or nutrient broths corresponding to the bacterial strains at concentrations of 1,250, 625, and 250 mg/L (final concentration: 625, 312.5, and 125 mg/L) which were not expected to exert any antibacterial activity and filter sterilized using 0.2 µm filter. The bacterial culture suspensions were adjusted to an optical density of 0.1 at 600 nm (OD600) and dispensed into a 96 well microtiter plate (100 µL) with or without SLCPP. After 24 h of incubation at 37°C, spent media were removed and the wells were washed twice with phosphate-buffered saline (PBS) (pH 7.2). The biofilms remaining on the surface of plates were stained using 0.1% (w/v) crystal violet for 10 min. Following staining, the wells were washed three times with distilled water and air dried. The bound dye was solubilized in 200 µl of 30% (v/v) ethanol. Then the OD600 was recorded using a microplate reader (Synergy™ 2, BioTek, Winooski, VT, USA). The percentage inhibition of biofilm formation for each concentration of SLCPP was calculated according to the following equation:
Nutritional components of the grower and finisher diets are shown in Table 1. The grower feed was set to have an apparent metabolizable energy (AMEn) of 3,100 kcal/kg and a crude protein (CP) content of 20.5% and the finisher diet had AMEn of 3,150 kcal/kg and CP content of 20% [26]. The basal diets were formulated according to the Korean Feeding Standard [26] for broilers to meet or exceed nutrients levels. In the polyphosphate containing diet, amount of SLCPP equivalent to 0.1% of the diet was dissolved in water (1 L) and then premixed with a small amount (2 kg) of basal diet manually. The premixed feed was then mixed with the entire feed using a feed mixer for 10 min (DKM-350SU, DAE KWANG, Hwaseong, Korea). The antibiotic containing diet was prepared by mixing basal diet with ENRACIN-10 (WooGene B&G, Seoul, Korea) that contains enramycin 10 g/kg at a final concentration of 0.01% using feed mixer as mentioned above. This experiment was performed in accordance with the Animal Care and Use Committee (KU21036) of Konkuk University (Seoul, Korea).
1) Mineral premix provided the following per kg of diet: Fe, 80 mg; Zn, 50 mg; Mn, 60 mg; Co, 0.3 mg; Cu, 10 mg; Se, 0.2 mg.
The experiment was conducted at Yonam College (Cheonan, Korea) during August to October, 2019. A total of 480 1-day-old male Ross 308 broiler chicks were raised in a group of 40 birds in metal pens (1.8 m × 1.8 m) at a stocking density of 20 kg/m2. The birds were randomly allocated to three dietary treatment groups: 1) basal diet without any additive (NC); 2) basal diet containing 0.01% ENRACIN-10 (PC); and 3) basal diet containing 0.1% SLCPP (SPP). Each treatment consisted of four replicates with 40 birds each. After 7-day of adaptation period on a basal diet, 40 birds of each pen were relocated to equalize their initial body weights among each replicate. Grower diet with or without additive was given from day 7 to day 21 (grower phase). After day 21, the finisher diets containing the same ratio of additives were used. The experiment lasted for 35 d (finisher phase). The temperature was maintained at 33 ± 2°CC for the first 7 days (adaptation period), and then it was gradually decreased by 2°C every week until 26°C was reached and maintained thereafter with 24 h of continuous artificial light exposure. Feed in mash form and drinking water were provided ad libitum during the whole period.
Body weight (BW) and feed intake (FI) were measured weekly, and mortality was checked daily. To measure BW, weights of all the birds within a pen were measured collectively and then average weight per bird for each pen was calculated. FI was calculated by subtracting the weight of the remaining feed from the weight of the feed offered every week. Body weight gain (BWG) was calculated as the difference between BW measured on each week and the initial BW, and was used to calculate average daily gain (ADG). Feed conversion ratio (FCR) was calculated as the ratio of FI to ADG.
At the end of the experiment, two birds were randomly selected from each replicate and killed by CO2 euthanasia. Immediately after blood sampling, the liver, spleen, bursa of Fabricius, small intestine (duodenum, jejunum, and ileum), and ceca were excised. The weights of liver, spleen, and bursa of Fabricius were measured using an electronic balance (EL4002, Mettler Toledo, Columbus, OH, USA) after removing fat and expressed as the ratio of weight per 100 g of live body weight. The intestine was divided into four sections: pancreatic loop (duodenum); from the end of the pancreatic loop to Meckel’s diverticulum (jejunum); from Meckel’s diverticulum to ileocecal junction (ileum); left and right ceca (ceca). The weight and length of each section were measured after removing their contents. The weight and length of the intestinal sections are expressed as relative values per 100 g of live body weight. Relative weight of the intestinal sections divided by the length was also obtained.
About 15 ml of blood was collected immediately through cardiac puncture after euthanasia into an ethylenediaminetetraacetic acid (EDTA)-treated blood collection tube, stored at 4°CC, and centrifuged at 1,500 rpm for 10 min using a centrifuge (HA-1000-3, Hanil Science Medical, Daejeon, Korea) to separate the serum. The separated serum was used to determine the levels of aspartate aminotransferase (AST), alanine aminotransferase (ALT), gamma glutamyl transferase (GGT), triglyceride (TG), total cholesterol (TC), uric acid (UA), glucose, total protein (TP), albumin, creatinine, blood urea nitrogen (BUN), calcium, and inorganic phosphorus using an automated biochemical analyzer (CHEM 7000i, Fujifilm, Tokyo, Japan).
After dissecting out jejunum, ileum, and ceca, they were immediately kept in ice and transported to the laboratory for microbial enumeration. Intestinal contents were collected aseptically into falcon tubes (50 mL) and the number of viable bacteria was enumerated within 24 h using standard agar culture method on deMan Rogosa Sharpe (MRS; Difco), nutrient, MacConkey (Difco), and Streptococcus thermophilus(ST) agar plates as described by Dave and Shah [27] with some modifications. Coliforms and lactose negative enterobacteria were counted on MacConkey agar. Total aerobic bacteria counts were obtained on nutrient agar. Lactic acid bacteria (LAB) were counted on MRS agar [28]. Streptococcusspp.were counted on ST agar. All contents of the intestine collected per treatment were sampled. Thereafter, 1 g of each sample was serially diluted with sterilized distilled water. Suspensions were then plated onto each medium and cultured for 24–48 h at 37°CC. After incubation, colonies were counted and expressed as Log CFU/g.
Data were analyzed in a completely randomized design using the PROC mixed procedure of SAS 9.4 (SAS Institute, Cary, NC, USA). For growth performance parameters, each pen was considered as the experimental unit. Each bird was considered as the experimental unit for the analyses of organ, blood, and intestinal microflora. All data were analyzed by one-way analysis of variance (ANOVA). The significance of difference among treatments was assessed using Tukey’s test at p-value ≤ 0.05. Results are presented as means and SEM.
RESULTS AND DISCUSSION
Antimicrobial activity of SLCPP against poultry bacterial pathogens is presented in Table 2. All pathogenic strains except S. entericaser. Gallinarum showed inhibition zones at different concentrations of SLCPP. At 10 mg/mL of SLCPP, only E. coli O157:H7, S. entericaser. Pullorum, and S. sonnei showed inhibition zones. However, L. monocytogenesshowed a very weak inhibition zone at 100 mg/mL of SLCPP only. The potential of the antibacterial activity of SLCPP decreased in the following order: P. aeruginosa> S. sonnei > S. entericaser. Pullorum > E. coli O157:H7 > K. pneumoniae> L. monocytogenes. Previously, polyphosphate has been reported to show antimicrobial activities against various Gram-positive bacteria [18,29]. The antimicrobial activities of polyphosphate against various Gram-positive bacteria is related to its ability to chelate divalent cations, resulting in cell division inhibition and loss of cell wall integrity [18]. However, relatively little attention has been paid toward the mechanistic effect of polyphosphate on Gram-negative bacteria. Previously, Obritsch et al. [30] have reported that generation time and lag phase of E. coli K-12, O157:H7, and S. entericaser.Typhimurium were increased while growths of B.subtilis, S. aureus, and L. plantarum were inhibited by the polyphosphate treatment. Contrastingly, in the present study, SLCPP demonstrated antimicrobial effects against tested Gram-negative poultry pathogens.
Pathogenic bacteria are known to live in the form of biofilm and show increased antimicrobial resistance than the planktonic cells [31]. Therefore, the effect of SLCPP on the biofilm inhibition was determined toward five poultry pathogens and is presented in Fig. 1. Previously, it has been indicated that percentage inhibition above 50% signifies good anti-biofilm activity [32]. SLCPP had varying degrees of activity on the prevention of biofilm formation ranging from 12% to 64%. Among the tested five strains, only K. pneumoniae and P. aeruginosa showed inhibition more than 50%, suggesting good anti-biofilm activity. In addition, the effect of SLCPP on inhibition of biofilm formation was concentration-dependent. This is the first study to determine the anti-biofilm activity of the SLCPP against poultry pathogens. The potential of SLCPP to inhibit formation of biofilms holds promise for reducing the attachment and colonization of poultry pathogens to host tissues.
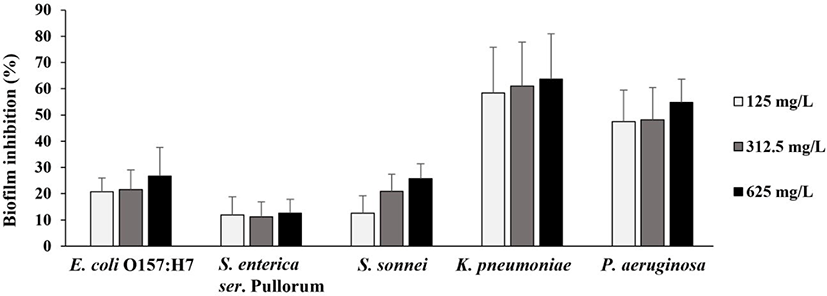
BW, BWG, FI, and FCR for each phase are shown in Table 3. On day 21, BW of PC and SPP groups were significantly higher than those of the NC group (p< 0.05). BWG, ADG, and FCR of grower phase were significantly improved in PC and SPP groups than in the NC group (p< 0.05). However, there was no significant change in FI (p> 0.05). Phosphorus plays an important role in cellular and membrane function, metabolism of fats and carbohydrates, and acid–base balance in poultry [33]. Several studies have used various forms of phosphorus minerals such as sodium phosphate, calcium phosphate, ammonium polyphosphate as sources of dietary phosphorus for broiler feed [34-37]. For instance, Jensen and Edwards [35] have reported that when 0.1% or 0.2% of ammonium polyphosphate was added as a phosphorus supplement to the broiler’s diet for 3 weeks, BW, FCR, and bone calcification were significantly improved. Another study [34] has reported the effectiveness of ammonium polyphosphate in drinking water as a sole source of supplemental phosphorus in broilers. Commercial poultry diets include several inorganic phosphates such as dicalcium phosphate (DCP), monocalcium phosphate (MCP), mono-dicalcium phosphate (MDP), defluorinated phosphate (DFP), and mono-sodium phosphate based on calcium to phosphorus ratio (Ca:P) to meet the mineral requirement for efficient growth of birds. These inorganic phosphates affectearly broiler performance, tibia mineralization, and Ca and P digestibility differently, which might be due to the variation in digestible minerals of specific inorganic phosphate [33]. Nevertheless, there are very few studies on long chain polyphosphates as growth promoting additives for livestock. According to the results of this study, feeding SLCPP could promote the initial growth of broilers compared to the NC. Its growth-promoting effect was of similar degree to antibiotic treatment. This might be due to the antimicrobial and anti-biofilm activity of SLCPP which was observed in our in vitro experiment. SLCPP might have also effectively acted on birds at their early growth stage by reducing their susceptibility to infections. Therefore, their initial growths were promoted as immunity might have been improved.
The relative weight of liver, spleen, and bursa of Fabricius are shown in Table 4. The dietary SLCPP did not affect the relative weight of liver which indicates that feeding SLCPP does not cause toxicities. Thymus, bursa of Fabricius, and spleen are the main originators of the immune cells involved in the cellular and humoral immunity in birds [38]. In this study, the relative weight of bursa of Fabricius remained unaffected among the treatment groups (p > 0.05). However, dietary SLCPP significantly increased the relative weight of spleen as compared with the enramycin fed birds (p < 0.05). Greater indices of spleen in birds might be determined as indicators of the augmented proliferation of immune cells, which implies better immunity [38]. However, it is not clear why broilers fed with antibiotic containing diet had lighter spleen weights. Moreover, the relative weight of spleen was not significantly different between SPP and NC groups (p> 0.05). This indicates that dietary supplementation of SLCPP had observed no adverse effects on the activity or function of immune organs compared to the NC group. Therefore, we speculate that SCLPP might help nutrients to be distributed well for the development of antibodies and immune organs required for immune activity. However, further studies are needed to ascertain this by measuring other immune parameters.
Table 5 shows the effect of dietary SLCPP on the relative weight and length of intestinal segments of broilers. The relative weight of duodenum did not show any significant difference among the dietary groups. However, its length significantly decreased in SPP and PC groups as compared with the NC group (p < 0.05). Regarding jejunum, its weight and length in the SPP group were not significantly different from other groups, although the PC group showed lower values than the NC group (p < 0.05). Likewise, the weight of ileum in SPP group was not different from that in the NC or PC group, although the PC group showed significantly lower value than the NC group (p <0.05). However, the weight or length of ceca remained unaffected among the treatment groups (p > 0.05). In addition, there was no significant difference in the ratio of weight to length of each intestinal segment among the treatment groups either. It has been previously indicated that longer and heavier intestinal organs need greater amount of energy to maintain them, thus adversely affecting the productivity of birds [39]. Erener et al. [40] have reported improved performance parameters and decreased intestinal lengths in broilers fed green tea extract than those fed a basal diet. Reis et al. [41] have also reported that dietary supplementation of B. subtilis (DSM 1729) culture solution improved the FCR and BWG of male broilers while decreasing the weights and lengths of duodenum, jejunum, and ileum. In this study, dietary SLCPP decreased intestine size to a certain extent in the SPP group. This may be explained by the fact that SLCPP might have improved early growth performance and increased energy requirement for increased weight gain instead of increasing energy required for the maintenance of intestinal organs in birds, leading to decreased length of intestine.
There was no significant difference in any blood biochemical parameters among treatment groups except for the content of BUN (Table 6). BUN was significantly lower in the SPP group than in the NC group. BUN is a biochemical indicator that shows dietary protein efficiency. Lower concentration of this indicator means higher availability of dietary protein with decreased urea synthesis and higher hydration in the liver [42,43]. Protein feeding exceeding nutrient availability can cause emission of nitrogen waste products in the form of uric acid in feces and BUN [44]. In this study, the lower concentration of BUN in the SPP group could be due to a high bioavailability of protein required for promoting body growth.
Calcium and phosphorous are essential nutrients for broilers. As main constituents of the skeleton, they play an important role in bone development and mineralization [45]. According to previous in vitro studies, polyphosphate (P65) increases calcification and differentiation of osteoblastic cells [17,46] and thereby might affect bone regeneration. However, in the present study, dietary supplementation of SLCPP neither affected serum levels of calcium nor phosphorus, which might be ascribed to the low concentration of SLCPP used in the broiler diet. In addition, SLCPP did not affect other blood biochemical parameters, indicating that SLCPP could be used as a safe feed ingredient without having adverse effects on broilers.
Table 7 shows changes of microflora in different intestinal sections of broilers. In the jejunum, counts of coliforms and lactose negative enterobacteria, and Streptococcusspp. in SPP group were significantly higher than those in the NC group (p < 0.05) However, LAB and total aerobes in the SPP group were not significantly different from that in the NC group. Notably, antibiotic treatment significantly decreased the LAB and total aerobes while increasing the coliforms and lactose negative enterobacteria as compared with the NC group (p < 0.05). In the ileum and cecum, dietary SLCPP did not affect the bacterial counts among the dietary treatments. However, the LAB count in the PC group significantly decreased than the NC group in the cecum (p <0.05). The balance of intestinal microflora is well known to play an important role in the health, growth, and productivity of poultry [47]. However, a consistent effect of dietary SLCPP on the microbiota harbored in the different sections of the intestine was not observed. Moreover, contrary to the initial expectation that dietary supplementation of SLCPP could inhibit coliforms and enterobacteria while increasing the beneficial microflora including LAB and Streptococcusspp. in broiler intestine, we did not observe such effect between the SPP group and the NC group in most of the intestinal segments. However, unlike antibiotics, dietary supplementation of SLCPP had no adverse effect on the LAB counts in the intestine of broilers. It is well known that LAB can exert beneficial effects by producing various antimicrobial substances such as organic acids and bacteriocins among many others. The presence of some lactobacilli in the intestine of chickens has been reported for regulating the composition of the intestinal microflora, developing immunity of the intestine, and promoting the health of chickens [48-50]. Additionally, it is necessary to consider the resistance of various intestinal microorganisms to SLCPP and thus, studies involving the changes in intestinal microflora after applying SLCPP at different dosages in the future are imperative.
CONCLUSION
In conclusion, SLCPP demonstrated antimicrobial and anti-biofilm activities against poultry pathogens in vitro. The dietary supplementation of extra inorganic phosphate in the form of SLCPP (0.1%) had increased growth performance parameters in the grower phase and reduced the length of the intestinal segments of broilers fed with a corn-soybean meal–based commercial diet containing 0.05% of phytase. Moreover, no adverse effects on the blood metabolites, immune organs, intestinal morphology, and counts of jejunal as well as cecal LAB were observed. However, the main drawback of this study is the smaller number of replicates and hence a large-scale broiler feeding experiment on the effectiveness of SLCPP as a potential growth promoter is imperative. Moreover, the implications of feeding different concentrations of SLCPP to determine its effect on bone quality and immune parameters in broilers should be studied further.