INTRODUCTION
Both supply and demand of feed ingredients are deteriorating due to climate change and increased demand for biofuel production. This has become a major concern to the domestic livestock industry in feedstuff-importing countries [1]. In order to resolve this problem, several attempts have been made to use agricultural by-products generated during agricultural food processing, and various studies have been conducted on utiliztation of by-products as a value-added feedstuff [1–5]. However, the digestibility of agricultural by-products by livestock is low. Fermentation is one processing method that can improve the digestibility [1,3,5,6].
Fermented feedstuffs are produced by inoculating microorganisms into digestible or non-digestible feedstock, which mainly comprises agricultural by-products [6]. The fermented feedstuffs act as probiotics because they contain beneficial microorganisms. Moreover, biological additives have attracted attention as antibiotic substitutes after the prohibition of antibiotic use in animals [7–10].
Several microbial species, such as Lactobacillus spp., Streptococcus spp., Bacillus spp., Clostridium spp., Bifidobacterium spp., Enterococcus spp., Saccharomyces cerevisiae, and Aspergillus oryzae have been utilized for fermentation of feedstuffs [11,12]. Among them, S. cerevisiae, Lactobacillus plantarum, and Bacillus subtilis are widely used as biological additives because of their beneficial effects. S. cerevisiae possesses a high oxygen affinity and hence stimulates the growth of anaerobic microorganisms in the rumen by increasing oxygen absorption in the gut [7,9,13]. It also stabilizes gastric and intestinal pH and increases animal weight gain by improving fiber digestibility [14–17]. It has been reported that probiotic feed reduces harmful microorganisms in the intestine, increases beneficial microorganisms by improving the gut microenvironment [12], enhances digestion and absorption of feed, and reduces odor emissions from manure [18,19]. Furthermore, S. cerevisiae has mycotoxin removal ability and can tolerate salivary and gastrointestinal environments [20]. Ethanol produced by S. cerevisiae accounts for a large portion of fermentation metabolites and increases the true metabolizable energy content [21] and feed palatability [22].
L. plantarum, a lactic acid bacteria (LAB), is one of the best suited lactic acid-producing species for decreasing feedstock pH [23], and thus can be implemented for extending shelf-life and decreasing nutritional loss in feeds [19]. Moreover, LAB with high intestinal adhesiveness block attachment of harmful organisms to the gut and repress the growth of noxious bacteria through production of antibacterial proteins [7–9]. In addition, they contribute to improved resistance to diseases and infections [24].
Bacillus spp. can survive easily because their spores are resistant to heat, light, and variations in pH, and the bacteria can provide protection against pathogens by producing antipathogenic substances [13,25]. Furthermore, Bacillus spp. have been shown to enhance amino acid absorption and reduce insoluble polysaccharides in feedstuffs [26].
To maximize the benefits of fermented feedstuffs, suitable fermentation conditions must be identified and optimized accordingly, based on microorganism species and incubation temperature and time. Korea, in particular, has four distinct seasons and the average daily temperature changes with each season. Temperature plays a critical role in fermentation, and thus, fermentation conditions should be changed according to the season. Currently, many studies on the types of microorganisms used in fermentation of feeds are ongoing, but studies on fermentation temperatures and duration are lacking [18,19,27]. Therefore, it is necessary to study culture temperature and time.
In this study, fermented feedstuffs were prepared by incubation with three microorganisms at different temperatures corresponding to the four seasons in Korea. Two types of experiments were performed. One experiment involved single-strain fermentation with S. cerevisiae, L. plantarum, or B. subtilis, and the other involved fermentation with all three strains. The changes in viable cell count of the inoculated microorganisms and concentrations of the metabolites produced during fermentation were analyzed at different temperatures to provide reference data for seasonal fermented feedstuff production.
MATERIALS AND METHODS
S. cerevisiae ATCC18824 was grown in yeast extract peptone glucose (2%) medium (YPD) (10 g/L yeast extract and 20 g/L peptone); the solid medium was prepared by adding 20 g/L bacto agar, and 100 μg/mL ampicillin (Amresco, Batavia, IL, USA) and 50 μg/mL kanamycin (Amresco, Batavia, IL, USA) were added to solid media for the detection of yeast in the mixed-strain fermentation. L. plantarum ATCC14917 was grown in De Man, Rogosa and Sharpe (MRS) medium; the solid medium was made by adding 20 g/L bacto agar, and 0.002 g/L sodium azide was added for the detection of LAB in the mixed-strain fermentation. B. subtilis ATCC6051 was grown on mannitol egg yolk polymyxin (MYP) agar as required. Precultured strains were grown overnight at 30°C and 250 rpm in shaking incubator.
In order to produce fermented feedstuffs, raw materials were prepared by mixing molasses, rice bran, crushed corn, and wheat bran at a ratio of 0.7:2:2:2.7, based on guidelines provided by the Korea Federation of Livestock Cooperative in Yeongcheon. The chemical compositions of the raw materials used are shown in Table 1.
Met, methionine; Lys, lysine; Arg, arginine; Thr, threonine; Leu, leucine; Ile, isoleucine; Val, valine; His, histidine; Phe, phenylalanine; Trp, tryptophan; ME, metabolized energy; Ca, calcium; P, phosporous; DM, dried matter; MC, moisture content; TDN, total digestible nutrients; CP, crude protein.
Rice bran, crushed corn, and wheat bran were mixed and pasteurized by autoclaving at 90°C for 15 min. Molasses was mixed with water. Water was then added to the raw materials (initial moisture content of 9.5%) to achieve an optimal moisture content of 45%. The density of the feedstuff was 0.7 kg/m3. The raw materials for manufacturing feedstuffs were suppliedby total mixed fermentation (TMF) feedstuff factory of the Korea Federation of Livestock cooperative in Yeongcheon.
S. cerevisiae (OD600 = 3.0), L. plantarum (OD600 = 1.0), and B. subtilis (OD600 = 2.5) were inoculated into the feedstuff at 0.5% weight. After mixing, 35 g of this mixture was added to a 50 mL conical tube to a density of 0.7 kg/m3 and then cultured at 10°C, 20°C, 30°C, or 40°C for 10 days. All experiments were repeated independently in triplicate, and variances are indicated with error bars.
To 140 mL of sterilized distilled water, 35 g of fermented feedstuffs was added, and the mixture was serially diluted to 10−2, 10−4, and 10−6 in sterilized water. Yeast colonies, which included the inoculated S. cerevisiae, were counted after plating on YPD agar containing ampicillin and kanamycin and incubation at 30°C for 48 h. The LAB colonies, which included inoculated L. plantarum, were counted after plating on MRS agar containing sodium azide and incubation at 37°C for 48 h. Bacillus spp. colonies, which included inoculated B. subtilis, were counted after plating on MYP agar and incubation at 37°C for 48 h. Colonies were counted to determine the numbers of viable microorganisms, and values are represented as colony-forming units (CFU)/g fresh matter.
Cell growth was monitored at an optical density (OD) of 600 nm using a spectrophotometer (OPTIZEN NANO Q, Neogen, Sejong, Korea). A 1 mL sample of fermented feedstuff was used to measure the pH (Seven compact™ pH/Ion meter S220, Mettler Toledo, Columbus, Ohio, USA) after a 10−1 dilution in sterilized water. Concentrations of lactic acid, acetic acid, and ethanol were detected using a high-performance liquid chromatograph (HPLC; Agilent 1260 series, Agilent Technologies, Santa Clara, CA, USA) equipped with a refractive detector and a Rezex ROA-Organic Acid H+ (8%) column (Phenomenex, Torrance, CA, USA). The column was eluted with 0.005 N of H2SO4 at a flow rate of 0.6 mL/min at 50°C.
RESULTS
Feedstuffs were inoculated with a single strain, and the number of viable cells was counted for each strain at 10°C, 20°C, 30°C, and 40°C in order to analyze the effect of fermentation temperature. When each strain was inoculated at 0.5% (g dry cells/g feeds), the initial viable cell counts were confirmed to be in the range of 4.4 to 6.7 Log CFU/g. Even though we inoculated a single S. cerevisiae, L. plantarum or B. subtilis strain for single fermentation, there was the possibility of mixed strains in the fermented feedstuffs because the material was pasteurized and not sterilized before inoculation.
The viable cell counts of each species increased and reached a peak within 3 days, except for those from the samples incubated at 10°C, and the cell counts gradually decreased after 3 days. At 20°C, the final viable cell counts of the three species were similar to the initial cell numbers (Fig. 1B). However, at 30°C, the viable cell counts of LAB declined below the initial cell number after day 7 (Fig. 1C). At 40°C, yeast and LAB cell numbers dropped significantly below the initial cell number, and only Bacillus spp. persisted at the initial viable cell count (Fig. 1D). However, at 10°C, cell growth patterns were quite different than those at the other temperatures. The viable cell counts of each strain were not markedly increased, and only Bacillus spp. viable cell numbers slowly increased after 5 days. Moreover, no viable cell counts of yeast were detected after 10 days (Fig. 1A), suggesting that 10°C was not suitable for the proliferation of all three microorganisms (yeast, Bacillus spp., and LAB).
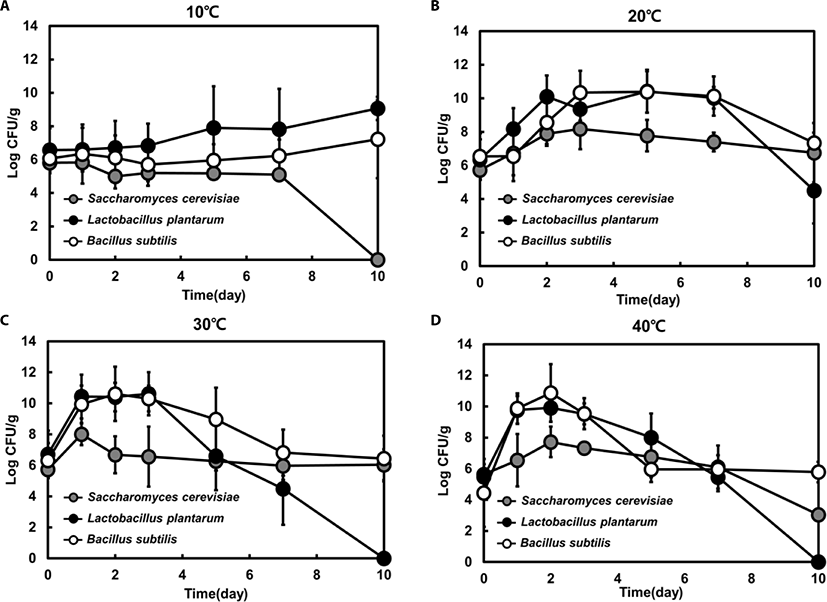
The LAB viable cell counts reached a peaked within 2 days and decreased steadily after 3 days at 20°C, 30°C, and 40°C. However, at 10°C, the viable cell number remained slightly increased after 3 days. LAB viable cell numbers reached the highest value of 10.4 Log CFU/g at 20°C (Fig. 1). Bacillus spp. showed a similar growth pattern to LAB at all temperatures. The viable cell counts of Bacillus spp. were nearly equal to those of LAB at 20°C, 30°C, and 40°C. However, Bacillus spp. maintained numbers of at least 6 Log CFU/g at all tested temperatures throughout the fermentation process, unlike LAB and yeast (Fig. 1). Although yeast numbers did not exceed 108 CFU/g at any temperature, yeast viability was sustained at 20°C and 30°C throughout the fermentation process. At 10°C and 40°C, the viable cell numbers decreased slowly, and no growth was detected at day 10 of fermentation at 10°C. These results reveal that incubation temperature affects the growth profile of this species.
It has been reported that yeast growth is inhibited by lactic acid produced by LAB [28–31]. In accordance with previous studies, our results also show that yeast numbers did not increase after the growth of LAB under all tested conditions (Fig. 1). Moreover, fermentation was completed by 7 days, at which point the cell numbers of all strains dropped sharply. Bacillus spp. strains showed increased proliferation at high temperatures, due to their heat-resistant spores [18,25].
Next, we analyzed mixed-strain fermentation and investigated the interaction among all three strains (yeast, Bacillus spp., and LAB) based on viable cell counts during feed fermentation at different temperatures. The fermentation pattern was changed after 3 days and the growth of most species was highest within 2 days.
There was no significant difference in growth patterns between single- and mixed-strain fermentation at 10°C and 40°C (Fig. 2). However, the proportion of each strain in the mixed-strain culture was different from that in the single-strain culture at favorable temperatures (i.e., 20°C or 30°C). Similar to the single-strain fermentation, LAB was the dominant strain at 10°C, 20°C, and 30°C in mixed fermentation until day 3 (Fig. 3A, B, and C). However, after day 3, the mixed-strain culture at 30°C was primarily comprised of yeast and Bacillus spp. (Fig. 3C).
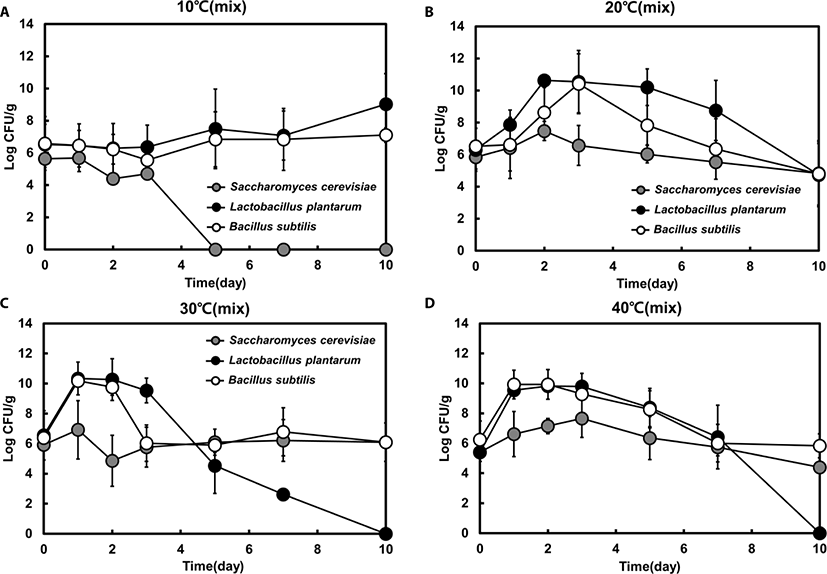
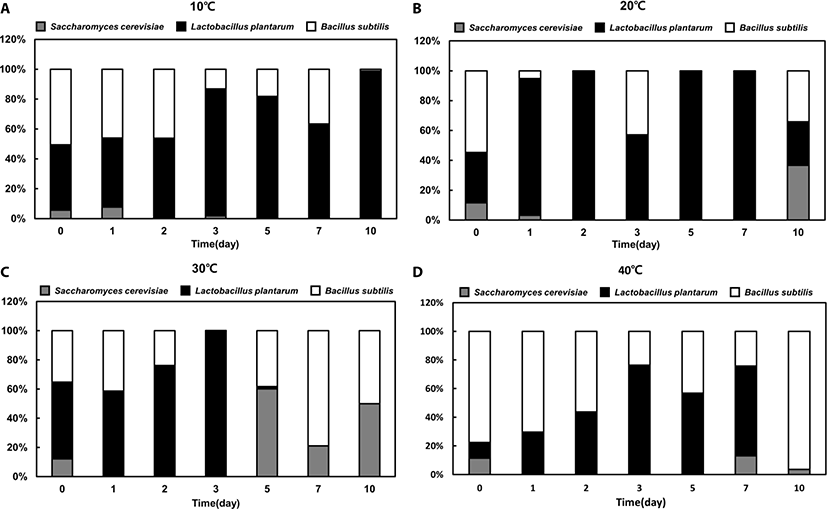
Bacillus spp. was the dominant species in the late phase of fermentation at 30°C and in the early phase of fermentation at 40°C, indicating that the portion of Bacillus spp. was dominant at higher temperatures (Fig. 3C and D). Although the portion of yeast were markedly increased from day 5 to 7 at 30°C in mixed fermentation, yeast was not the dominant species in mixed fermentation.
For both single and mixed fermentation at 10°C, 20°C, 30°C, and 40°C, we monitored pH levels (Fig. 4). The initial pH of the feedstuffs was approximately 6.5, and the pH dropped instantly or gradually to about pH 4.3 at all tested temperatures. Once the pH decreased to approximately 4.3, the pHs were not further changed. At 40°C, all feedstuffs reached pH 4.3 in the early stage of fermentation (day 1), but at 10°C, the pH had barely reached 4.3 in the last stage of fermentation (day 10) (Fig. 4). Moreover, the pH level was maintained in single- yeast fermentation at 20°C, while the pH declined significantly in single LAB and Bacillus spp. fermentations after 3 days. Interestingly, the pattern of pH level in the mixed fermentation was similar with that of the single LAB strain fermentation, suggesting that LAB was the dominat strain (Fig. 3).
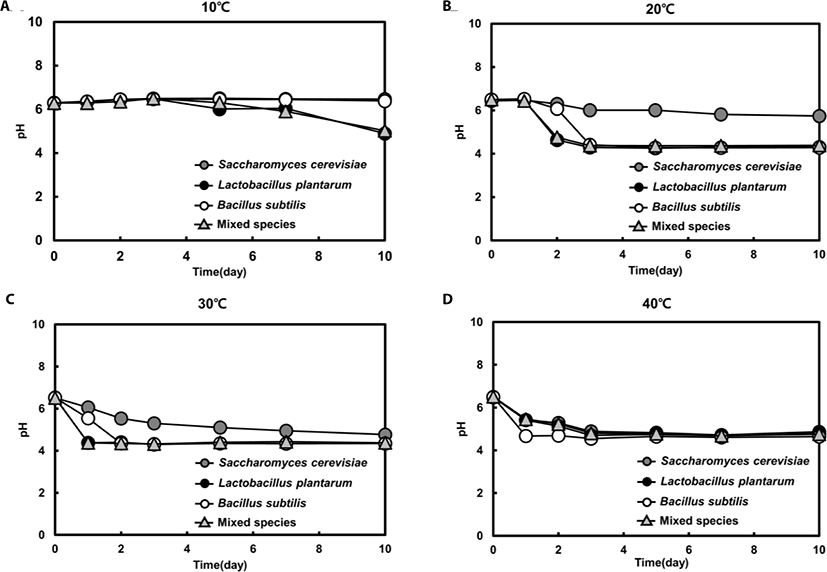
The decrease in pH may be attributed to the production of organic acids such as lactic acid, acetic acid, and propionic acid, as reported in previous feed fermentation studies [19,23]. The maintenance of a pH level of 4.3 can be explained by LAB utilization of sugars originating from hemicellulose breakdown, causing a decrease in pH [23].
To cleary identify optimal fermentation conditions, we also analyzed the changes in levels of metabolites such as lactic acid, acetic acid, and ethanol, which are generally used to assess the quality of fermented feedstuffs [32–38], in single- and mixed- culture fermentation.
Lactic acid, which is one of the representative organic acids of fermented feedstuffs, was produced in the early stages of both types of fermentation at 20°C, 30°C, and 40°C. The highest lactic acid concentration was 11.1 and 8.9 g/L for single and mixed fermentation at 20°C, respectively (Fig. 5A and B). On the other hand, at 10°C, lactic acid was produced after 5 days, and the concentration was very low (2.7 g/L) in both the single and mixed fermentation (Fig. 5A and B). The overall results show that lactic acid production in mixed fermentation was relatively lower than that in the single-strain fermentation.
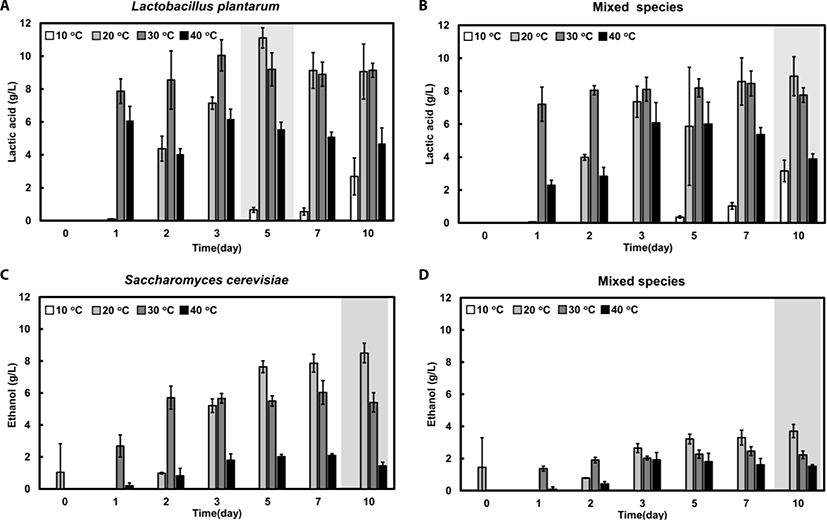
The level of ethanol increased constantly during the fermentation process in the single and mixed fermentations at 20°C, reaching a maximum level of 8.5 and 3.7 g/L, respectively (Fig. 5C and D). At 30°C, the level of ethanol was not significantly changed after day 2 (5.4–6.0 g/L). Ethanol was not produced at 10°C and only slightly produced at 40°C in both fermentations (Fig. 5C and D). The overall results indicated that ethanol production was substantially higher in single-strain fermentation than in mixed-strain fermentation at all tested temperatures. In addition, the effect of temperature on ethanol production was much greater in single-strain fermentation than in mixed-strain fermentation.
Acetic acid production was highest at 40°C, and there was no difference between acetic acid levels of the single-strain and mixed-strain fermentation (data not shown). With regards to the concentration of metabolites such as organic acids and ethanol, single-strain fermentation was found to be more advantageous than mixed-strain fermentation (Fig. 5B and D).
The results reveal that the optimal fermentation conditions for maximal lactic acid and ethanol production were single-strain fermentation at 20°C for 7 days. The representative results of the metabolite concentrations and viable cell numbers at day 7 are shown in Table 2. Moreover, our results show that more time is required to maximize the benefits of fermented feeds incubated at lower temperatures. At low and high temperatures, yeast growth and metabolism is inhibited [39–41]. The higher level of metabolites in single-strain fermentation, as compared to mixed-strain fermentation, could be caused by the competing interaction between the species in the mixed-strain fermentation environment [28,42].
DISCUSSION
There are no precise guidelines or standards for the fermentation of feedstuffs, and many studies have been conducted to optimize fermentation conditions. Among the various parameters used to estimate feedstuff quality, pH is an important parameter because various organic acids are produced by microorganisms during fermentation [43]. A pH value of 4.0 usually indicates that fermentation has been completed efficiently [18,44]. A low pH prevents growth of harmful organisms [42] and increases production of rapidly degradable carbohydrates [45]. In addition, viable cell count is an important parameter for fermented feedstuffs, as it indicates the ability of a culture to sustainfermentation, and animals benefit from ingestion of the microorganisms [46]. The use of probiotics increases beneficial gut microorganisms and reduces the number of harmful organisms in the intestine of ruminant animals [33]. Moreover, absorption and digestion are improved [47,48], and odor emissions from manure are decreased [44]. The S. cerevisiae, L. plantarum, and B. subtilis strains used in this study also function as probiotics.
Furthermore, organic acids such as lactic acid and acetic acid inhibit the growth of pathogenic bacteria [42,48] and enhance mineral and nutrient utilization [49]. Organic acid production stim-ulates chewing rate and helps eliminate total hydrogen ions of gastric and intestine [50]. Ethanol stimulates appetite and improves palatability, which promotes weight gain [3].
Comprehensively, there were no major changes in metabolic parameters, such as organic acidand ethanol production, and the number of viable cells decreased rapidly after 7 days. Hence, the optimal fermentation time for feedstuffs is considered to be 7 days. Considering that the fermentation method requires modification in Korea according to the season in order to maximize theeffects of fermented feeds, the four temperature conditions in this study correspond to the fourseasons: 20°C and 30°C conditions represent spring, fall, and early summer temperatures, the 10°C condition represents winter temperature, and the 40°C condition represents summer.
This research provides fermentation data based on different temperatures corresponding to the four seasons in Korea and hence, can serve as a potential guideline for fermentation of feedstuffs. Byusing our results as a reference, the specific fermentation times required to produce fermented feedstuffs can be adjusted based on individual preferences between viable cell density and metabolites,such as organic acids. Furthermore, our findings contribute to building baseline data for fermented feedstuffs.